Premium Only Content
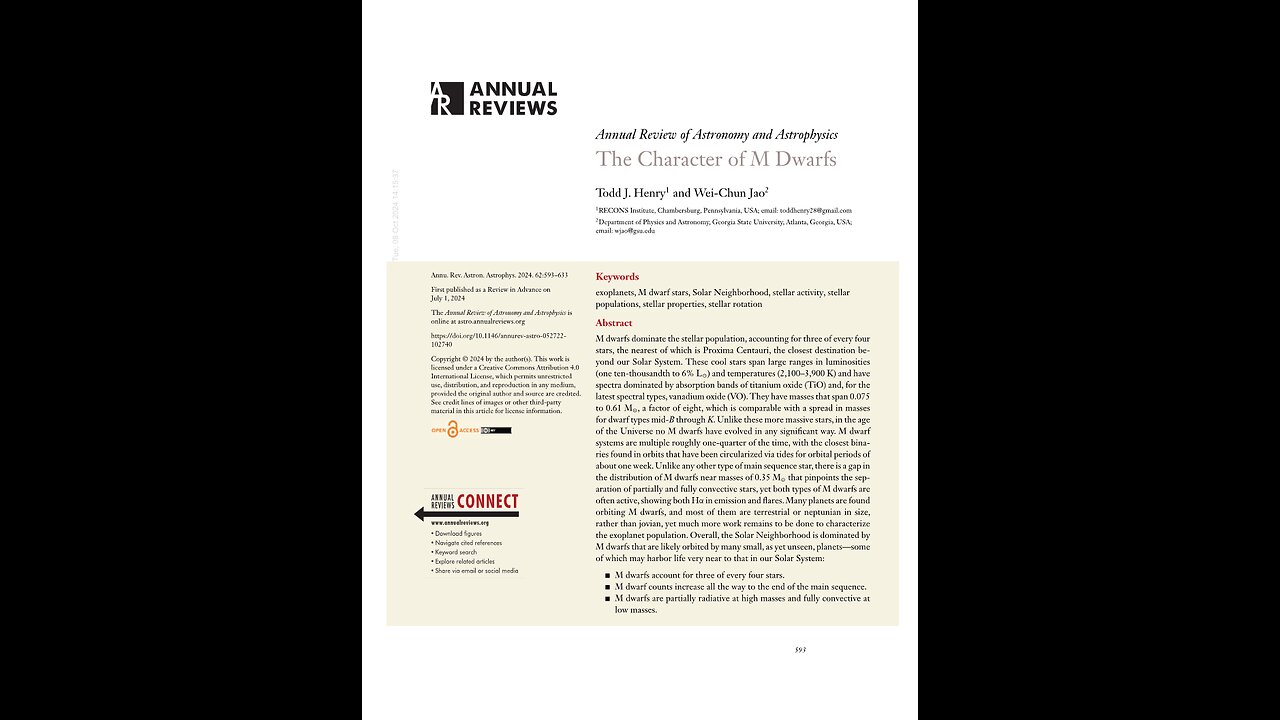
The Character of M Dwarfs. Todd Henry, a Puke (TM) Audiopaper
Index of Other Science Articles:
https://rumble.com/v3t4yzj-index-of-science.-music-by-dan-vasc.html
Annual Review of Astronomy and Astrophysics
The Character of M Dwarfs.
Todd Henry and Wei-Chun Jao.
RECONS Institute, Chambersburg, Pennsylvania, USA
Department of Physics and Astronomy, Georgia State University, Atlanta, Georgia, USA.
Online at astro.annualreviews.org
https://doi.org/10.1146/annurev-astro-052722-102740
Keywords: exoplanets, M dwarf stars, Solar Neighborhood, stellar activity, stellar populations, stellar properties, stellar rotation.
Abstract.
M dwarfs dominate the stellar population, accounting for three of every four stars, the nearest of which is Proxima Centauri, the closest destination beyond our Solar System. These cool stars span large ranges in luminosities, one ten-thousandth to 6 percent of Solar Luminosity and temperatures, 2,100 to 3,900 Kelvin and have spectra dominated by absorption bands of titanium oxide, T-I-O, and, for the latest spectral types, vanadium oxide (VO). They have masses that span 0.075 to 0.61 Solar Masses, a factor of eight, which is comparable with a spread in masses for dwarf types mid-B through K. Unlike these more massive stars, in the age of the Universe no M dwarfs have evolved in any significant way. M dwarf systems are multiple roughly one-quarter of the time, with the closest binaries found in orbits that have been circularized via tides for orbital periods of about one week. Unlike any other type of main sequence star, there is a gap in the distribution of M dwarfs near masses of 0.35 Solar Masses that pinpoints the separation of partially and fully convective stars, yet both types of M dwarfs are often active, showing both H alpha in emission and flares. Many planets are found orbiting M dwarfs, and most of them are terrestrial or Neptunian in size, rather than Jovian, yet much more work remains to be done to characterize the exoplanet population. Overall, the Solar Neighborhood is dominated by M dwarfs that are likely orbited by many small, as yet unseen, planets, some of which may harbor life very near to that in our Solar System:
M dwarfs account for three of every four stars.
M dwarf counts increase all the way to the end of the main sequence.
M dwarfs are partially radiative at high masses and fully convective at low masses.
One. Introduction.
Hertzsprung-Russell diagram (HRD): a plot showing the distributions of stellar luminosities and effective temperatures.
O-B-A-F-G-K-M: letters used to classify spectral types of stars based on features in spectra.
M dwarf stars, often called red dwarfs, dominate the population in the Solar Neighborhood, and presumably throughout the entire Milky Way and other galaxies, accounting for three of every four stars. Red dwarfs are so intrinsically faint that even though many of them are close by, they are beyond the reach of the naked eye, the brightest red dwarf, Lacaille 9352, has a V magnitude of 7.34, and only four red dwarfs are brighter than V equals 8. Thus, M dwarfs comprise a stellar swarm that lurks unseen, surreptitiously dominating the Galaxy.
These relatively small, unassuming stars are found in the lower right hand corner of the fundamental map of stellar astronomy, the Hertzsprung Russell diagram (HRD), along which the main sequence stars stretch through O-B-A-F-G-K-M dwarfs. Compared to our G dwarf Sun, M dwarfs are smaller in diameter by factors of about two to more than ten and have masses comparably smaller.
They span a factor of eight in mass, a broader range than the A-F-G-K type stars combined, and although relatively low in mass and small in size, M dwarfs nonetheless provide more mass to our Galaxy than any other single spectral type because of their large numbers.
Their photospheres are roughly only one-half the temperature of the Sun’s, and they are two to four orders of magnitude less luminous. Although the Sun will live roughly 10 billion years, not a single M dwarf has evolved in any meaningful way since its birth over the 13.7 billion years of the Universe’s existence.
As they continue to form in great numbers in the thin disk of the Milky Way, they also populate the thick disk and halo as effectively pristine, unchanged versions of their original selves. M dwarfs are lonelier than other stars, with only about one in four systems having more than one stellar component, in contrast to more massive stars that are in multiples 40 to 100 percent of the time.
There are, in effect, three types of stars all collected into one spectral type. M dwarfs with masses of 0.4 to 0.6 Solar Masses have partially radiative, partially convective interiors and generally behave in ways similar to their larger K dwarf cousins. Those with masses of 0.3 to 0.4 Solar Masses lie in a transition zone between partially and fully convective stars and have interiors that shift in structure unlike any other type of main sequence star. The smallest M dwarfs with masses from 0.3 Solar Masses down to the stellar substellar limit at 0.075 Solar Masses are fully convective and have molecules in their photospheres, including T-I-O, V-O, and H-2-O. Overall, M dwarfs create a wide range of astrophysical characteristics worthy of study and create various types of environments for the many planets that have been found over the past few decades to orbit them.
This presentation is primarily observational in nature, as the many theoretical considerations worthy of attention would expand the article into a very long endeavor. The last treatment focused on very low-mass stars in the Annual Review of Astronomy and Astrophysics (ARAA) was the article by Liebert and Probst in 19-87, which did a superb job of summarizing our knowledge of the smallest stars at the time, with particular highlights including masses for M dwarfs and considerations of just how many very low-mass stars there are in the Milky Way. A comprehensive overview of small stars can be found in the book New Light on Dark Stars by Reid and Hawley two thousand and five. Nearly four decades since the last ARAA article, we have learned much about these common denizens of the Solar Neighborhood. In this review, we explore many facets of M dwarfs, from their discovery, Section 2, and the definition of what it means to be an M, red dwarf, Section 3, to various population aspects, Section 4. We probe beyond their photospheres to consider the internal structures of M dwarfs, Section 5, and the consequent activity seen on their surfaces, Section 6, presumably driven by events in their interiors. We then explore the exoplanets currently posited to be orbiting the nearest M dwarfs, Section 7, before summarizing what we know about the most common stellar residents of our Galaxy, Section 8.
Two. DISCOVERING M DWARFS.
Two Point One. The First M Dwarfs Found.
Pinning down precisely when a star was “discovered to be an M dwarf” is rather difficult because the assignment of M dwarf as a spectral type did not occur until the twentieth century, though these small stars were certainly noted much earlier. For example, M dwarfs are not included in the spectral atlas of Morgan et al from 19-43, although they presented many M-type giants and subgiants. Likely the first M dwarf cataloged, before the M type had been invented, was Lacaille 93-52, G-J 887, M 1 dwarf, at 3.3 parsec, or 100 Peta-meters, it is the tenth nearest star system. The star was observed by Lacaille in 17-52 and measured in 18-81 to have a high proper motion of nearly 7 arc seconds per year by Gould in 18-81. At least two intrinsically fainter stars were identified in the nineteenth century. Lalande 21-18-5, G-J 411, M 1 dwarf, at 2.5 parsecs, 77 Peta meters, the fourth nearest, was first cataloged by Lalande in 18-01 and is the brightest M dwarf in the northern sky at V equals 7.53. It was noted to be a high proper motion star by Argelander in 18 57, Lynn 18-72, and was listed as type “Ma” by Adams and Kohlschutter in 19 14, presumably indicative of an M dwarf type. Groombridge 34, GJ 15 A, M 1 dwarf, at 3.6 parsecs, one hundred and eleven Peta meters the sixteenth nearest, was discovered by Groombridge in 18 38 and was also noted as type “Ma” by Adams and Kohlschutter 19 14.
RECONS: Research Consortium On Nearby Stars.
The three nearest M dwarfs are Proxima, Barnard’s Star, and Wolf 359, each of which has a brief dossier in the Supplemental Text of the Supplemental Material. All three of these were discovered in the nineteen tens and remain among the most famous stars known. Proxima is the tertiary in the Alpha Centauri system. Barnard’s Star, GJ 699, M 3.5 dwarf, at 1.8 parsecs, 56 Peta-meters, the second nearest, was discovered by Barnard in 19-16 and remains the fastest moving star in the sky at 10.4 arc seconds per year. Wolf 359, GJ 406, M 5.5 dwarf, at 2.4 parsecs, 74 Peta meters, the third nearest, was also found because of its large proper motion, 4.8 arc seconds per year by Wolf in 19-19. Within the next decade, Ross in 19-26 provided a list of proper motion stars that included three M dwarf gems very near the Sun: Ross 128, GJ 447, M 4 dwarf at 3.4 parsecs, 105 Peta Meters, the eleventh nearest, Ross 154, GJ 729, M 3.5 dwarf, at 3.0 parsecs, 93 Peta-meters, the seventh nearest, and Ross 248, GJ 905, M 4.5 dwarf at 3.2 parsecs, 99 Peta Meters, the eighth nearest, all with proper motions larger than 0.7 arc seconds per year.
Six of the nine stars mentioned here are listed as type “dM” or “sdM” among the 181 stars studied by Joy in 19-47, whose work focused on spectral types and radial velocities of A-F-G-K-M dwarfs.
A figure in that paper provides a compelling Russell diagram illustrating M dwarfs on the lower main sequence, and is one of the first references that clearly highlights the nature of these low-luminosity red stars as a group and assigns them the letter M.
Section two point two GJ 10-61, A Classic Discovery Tale.
As time passed, larger and more sophisticated scans of the skies revealed great numbers of M dwarfs. The monumental sky surveys of Giclas et al in 19-71 and Luyten in 19-79 together searched the entire sky to photographic magnitudes of 16.5 for stars with proper motions greater than 0.2 arc seconds per year, most of which were red dwarfs. An example that builds upon that work is the classic discovery tale of GJ 10-61 (LHS 1565, M 5 drawf-e, 3.7 parsecs, 115 Peta Meters, found to be the twentieth nearest stellar system 30 years ago. This star retains that rank among stellar systems today, making it the nearest M dwarf found in three decades, even in the age of the all-sky astrometric surveys of Hipparcos and Gaia, see Section 4 point 1.
As part of an effort to reveal primarily M dwarfs near the Sun that had not yet been identified, the REsearch Consortium On Nearby Stars, RECONS, team targeted GJ 10-61, V equals 13.09, and three other potential nearby stars based upon photometric parallaxes that placed all four stars nearer than 5 parsecs, 154 Peta-meters. However, optical spectroscopy revealed the others to be distant giants, leaving GJ 10-61 as the lone probable nearby star, with a spectrum virtually identical to Proxima’s. The final datum confirming the star to be a solar neighbor came from the long-term astrometric work carried out at Siding Spring Observatories, for example Lanna and Bessell 19-86, which provided a parallax of 273.4 plus or minus 5.2 milli arc-seconds, 3.7 parsecs, 115 Peta-Meters, thereby proving the star to be less than three times further away than Proxima. This is a classic example of the discovery process for nearby M dwarfs that began with photometric efforts, followed by spectroscopy efforts, and ultimately proven to be nearby via astrometric work that provided the trigonometric parallax.
Three. DEFINING M RED DWARFS.
Three point one. The Brightest and Hottest to the Faintest and Coolest.
M dwarfs have historically been identified using spectra, for which details are given in Section 3 point 2.
The brightest, hottest M dwarfs are virtually identical to the latest-type K dwarfs, whereas the faintest, coolest red dwarfs are in fact not assigned the letter M. Our understanding of stellar physics has advanced significantly in the past century, and though the spectral type M has been maintained for most red dwarfs, here we extend a bit further to the important astrophysical dividing line between stellar and sub stellar objects. Hence, throughout this article, when “M dwarf” is used we in fact mean “red dwarf,” which spans spectral types M 0.0 dwarf through L 2.5 dwarf.
Hydrogen burning limit: the critical mass required to start nuclear fusion.
Electron degeneracy pressure: a pressure from fermions that counters gravity seen in both brown and white dwarfs.
This is warranted because the smallest stars assigned the letter “L” are very similar to the slightly more massive and larger types assigned M 9 Dwarf or M 9.5 Dwarf, and we would be remiss if we ignored a few of the faintest, coolest red dwarfs. L dwarfs are separated from M dwarfs by diminishing T-I-O and V-O band strengths while metallic hydride and neutral alkali metal lines appear, yet nearly all objects with types through L2.5, except for a few young brown dwarfs, are, in fact, stars.
In contrast to these nuances of letters used for spectral types, absolute magnitudes are clearly defined and often superior when evaluating populations because photometry and parallaxes are now available for far more stars than are high-quality spectra. Furthermore, photometric measurements are usually made using carefully characterized filter sets, whereas spectral types are assigned using different wavelength windows and resolutions by different surveyors. Other advantages of photometric evaluations are the cases of closely separated components in which magnitudes combined with magnitude difference measurements make it possible to characterize components for which individual spectroscopic measurements are difficult, if not impossible, to obtain.
Here, we define red dwarfs by utilizing photometry and parallaxes in concert with spectroscopy to link the characterization to more familiar spectral types. To pin down the bright and faint limits for red dwarfs, we use absolute G magnitudes from Gaia Data Release 3, hereafter GDR3, Gaia Collaboration. This choice has been made for several reasons:
(a) millions of M dwarfs now have measurements at G, far more than the classic V magnitudes measured from the ground,
(b) Gaia provides G band measurements for close multiples down to separations less than an arc second in many cases, so individual components can be evaluated, and
(c) Gaia BP and RP datasets miss a few percent of stars that have G measurements, so G yields more complete samples.
The three panels of Figure 1 illustrate observational HRDs for objects within 25 parsecs, 771 Peta Meters. Absolute G magnitudes (MG) and G-K’s colors are used as proxies for luminosities and temperatures, respectively, where the Two-Micron All Sky Survey (2MASS) K’s magnitudes all have quality code A and objects have been matched to Gaia sources using the GDR3-2MASS best neighbors table. Defining red dwarfs requires that two lines be drawn in this HRD, one each at the high and low-mass, luminosity, temperature ends.
To delineate between K and M dwarfs at the high-mass end, we have collected spectral types from reliable, large surveys of nearby stars, including stars within 40 parsecs, 1.23 Exa-meters, the Palomar, Michigan State University survey, and the RECONS group. Because of differences in methodologies and the typical one spectral class errors in typing near this border, the resulting overlap between late K and early M types is evident in Figure 1b. We split the difference here, with roughly equal numbers of interlopers on either side, to set the cutoff for the highest-mass red dwarfs at MG equals 8.1.
To separate the smallest stars from the most massive brown dwarfs is rather more subtle than simply selecting a line, as was done between K and M types. Here, we rely on the work of Dieterich et al from 2014, who identified the hydrogen burning limit by using two intersecting characteristics of low-mass stars and brown dwarfs.
As mass is removed from a star, its radius shrinks because nuclear fusion rates in its core decrease, whereas as mass is removed from a brown dwarf, its radius expands because electron degeneracy pressure weakens. Thus, there is an inflection point in radius at the border between the lowest mass stars and highest-mass brown dwarfs, which Dieterich et al in 2014, found to occur at around 0.086 Solar Radii, log Luminosity over Lof Solar Luminosity around minus 3.9, and Effective temperatures around 2075 Kelvin. These attributes point to the lowest-mass stars having a spectral type of L2.5 dwarf. In Figure 1c, we use presumably single objects with spectral types from Best et al in 2020 and plot M dwarfs and L dwarfs, where the latter are a mixture of stars and brown dwarfs. The selected demarcation line at MG equals 17.8 is drawn where there is an obvious gap in the distribution, and below which the distribution of objects expands, as expected for brown dwarfs having a mix of masses, ages, and atmospheric properties.
It seems unlikely that there
Figure One.
Three different views of the observational Hertzsprung Russell diagram used to categorize red dwarfs are shown. Panel a captures objects within 25 parsecs from Gaia Data Release 3 having parallax errors less than 0.1 percent, where red dwarfs are represented by red points. White dwarfs are shown with open circles, and the Sun is marked as a yellow point for context near the top, where the vertical axis has been restricted to highlight red dwarfs. Panel b shows the adopted division between stars with spectral types of K (black) and M (red) at MG equals 8.1. Panel c shows the adopted hydrogen burning limit separating the lowest-mass red dwarfs from brown dwarfs at MG equals 17.8 for objects with spectral types of M (red) and L (black). Note that some L dwarfs are stellar red dwarfs. The points in panels b and c have been carefully vetted to be singles to the extent that is currently possible. Data used to make these three plots are available in the Supplemental Material.
This division also happens to be the location at which all L 2.5 dwarf types are above the line, so we select this line to mark the end of the stellar main sequence. There may be a small number of young brown dwarfs above this line, but there should be no stars below it. Age considerations also support the existence of a gap. For a set of objects with ages 0 to 10 Giga-years in the Solar Neighborhood, nearly all of the lowest-mass stars have collapsed to minimal stable radii and MG values, whereas only a small fraction of the youngest, most massive brown dwarfs will remain “puffy” and have similar MG while most have already faded to fainter than MG equals 17.8.
Table One. Table of red dwarf characteristics with a comparison to the Sun.
Masses, Luminosities Effective temperatures, Magnitudes in the G, V, I and K bands and the colors V minus I, and G minus K.
Dupuy and Liu in 2017 speculated that the hydrogen burning limit may be somewhat cooler than described by Dieterich et al. (2014) owing to possible systematic errors in temperatures derived from model atmospheres. However, using a macro set of more than 300,000 sources within 100 parsecs, 3 Exa Meteres, the Gaia Collaboration found a dip in the luminosity function at MG equlas 17.6, which is similar to our line at 17.8. Furthermore, evolutionary models of low-mass objects near the end of the main sequence show rapid temperature and luminosity drops in the first 1 to 2 Giga-years, which is consistent with the empirical gap seen in Figure 1. Recent research by Chabrier et al in 2023 focuses on a new equation of state for dense hydrogen helium mixtures. Although the results do not precisely match the MG and G K color values illustrated in the empirical plot in Figure 1, the overall trend traces the distribution of points. Their smallest star with mass 0.075 Solar Masses effectively settles on the main sequence after 3 to 4 Giga years, whereas brown dwarfs with masses of 0.074 and 0.073 Solar Masses continue to fade, thereby crossing the gap where the line of demarcation has been drawn. Thus, models continue to progress in refining the theoretical definition of the hydrogen burning limit, and the data shown in Figure 1 provide insightful constraints and a useful guide to the empirical end of the main sequence. We conclude that the HRD gap at MG equals 17.8 represents the bridge crossed by brown dwarfs as they cool and grow fainter, slipping down through and eventually off the main sequence of stars.
Table 1 provides general guidelines of red dwarf characteristics, including the high and low mass ends at M 0 dwarf and L 2.5 dwarf, as well as intermediate comparison points at M 3 dwarf, M 6 dwarf, and M 9 dwarf and values for the Sun for context. The values listed in Table 1 are based upon the RECONS spectral classification system, which is somewhat redder than others at early types, Section 3 point 2. The result is that for a given type like M 3 dwarf, the values assigned are lower in mass, fainter, cooler, and redder than in other spectral typing systems, although all values are self-consistent within this system. Overall, it is essential to keep in mind that because of the width of the main sequence, these are guidelines and that sets of stars assigned a specific spectral type will vary in the other characteristics listed in Table 1.
Section Three point two. Spectral Classification: Main Sequence M Dwarfs.
In the late nineteenth century, the “M” spectral designation was first used by Williamina Fleming and a team of women known as the Harvard Computers when they classified spectra for stars in Henry Draper’s catalog. The M type was propagated into the Harvard spectral classification system by Annie Jump Cannon, who added suffixes “a,” “b,” and “c” to indicate increasing T-I-O band strengths, a concept that has remained to the present for subtypes of the M class. During the IAU, International Astronomical Union, meeting in 19-22, the subtypes of M0 through M10 were introduced using T-I-O band strengths, and the letter “e” was added to indicate Balmer line emission, notably for H alpha.
With improving fidelity in spectral classifications, Joy in 19-47 introduced half-types for M dwarfs, and henceforth, assigning M dwarf types to half units has become the norm, with errors typically quoted to plus or minus 0.5 types.
Unfortunately, Joy’s classifications lacked spectral standard stars, so spectral types for M dwarfs from low-dispersion photographic plates remained somewhat subjective. The spectral standard stars published by Morgan et al in 19-43, could have potentially reduced such problems, but in their atlas, the M type stars only included giants and super giants for types M0 to M4, and no dwarfs.
A few M dwarf spectral standards were presented by Johnson and Morgan in 19-53, but only for M0 to M2 types that used T-I-O band strengths in the blue window, less than 6,500 Angstroms established by Morgan in 19-38, and Kuiper in 1942 to classify M dwarfs and to indicate effective temperatures.
Boeshaar in 19-76 extended M dwarf classification spectra to 6,800 Angstroms to include additional atomic and molecular lines, adding T-I-O bands, the V-O feature at 5,736 Angstroms, and Ca-O-H at 5,530 Angstroms, as well as a specific measure of the line ratio of V-O at 5,736 Angstroms over the T-i-O band at 5,759 Angstroms.
An important aspect of her classification system is that it was established by following the effective temperature trend from a set of M giant spectral standards. The Boeshaar system covered types M0 dwarf to M 6 dwarf, stopping before the end of the sequence primarily because the low effective temperatures of late type M dwarfs shifts the peaks of their spectral energy distributions into the near-IR, a 2,900 Kelvin M dwarf’s spectrum peaks at 1 micron, and the minimal flux at blue wavelengths made classifying the coolest M dwarfs difficult. The sequence was extended to types M 9 dwarf by Kirkpatrick et al in 19-91 using spectra that reached to 9,000 Angstroms, taking advantage of the redder limit to reach wavelengths at which the lowest temperature M dwarfs emit significant flux.
In general, the key aspects that separate late K dwarfs from early M dwarfs are that:
(a) The T-I-O bands are stronger for M dwarfs;
(b) The Ca-H and Mg-H lines are weaker in M dwarfs;
(c) The H alpha line appears in absorption in most K dwarfs, whereas it is often in emission for M dwarfs; and
(d) V-O absorption features are present for dwarfs later than type around M 5.0V dwarf.
Figure 2b illustrates an M dwarf spectral sequence for types M0 dwarf through M9 dwarf, with key T-I-O and V-O features used for classification highlighted, values for plotting the spectra are given in the Supplemental Material.
The advent of CCD’s, charge-coupled devices, for spectroscopy in the nineteen nineties made it also possible to use the spectral slopes evident in Figure 2 for classifying M dwarfs, and these slopes, in combination with differences in Potassium One, Sodium One, and Calcium Two line strengths, can be further used to distinguish M dwarfs from giants. Various groups have used these features and slopes to assign spectral types for great numbers of M dwarfs. The extensive dataset amassed for the nearest M dwarfs by the RECONS team enabled the development of an algorithm called ALLSTAR, to classify M dwarfs. A full set of RECONS standard stars with spectral types assigned for each 0.5 subtype from M 0 through M 9 dwarf is given in the Supplemental Material.
These stars have been selected to be among the brightest representatives of their types: M 0 to M 5 dwarf stars all have V less than 15 and M 5.5 to M 9 dwarf stars have V equals 13 to 19. All but two lie between DECLINATION plus 30 and minus 30, so they can be observed from most observatories in both the Northern and Southern Hemispheres. To take advantage of the large set of M dwarf spectra obtained during the Sloan Digital Sky Survey (SDSS), established template spectra of M dwarfs, M 0 to L 0 dwarf using polynomial relations discussed by Reid et al in 1995, and spectral features such as Ca H one, Ca H two, Ca H three, and T-I-O Five marked in Figure 2.
Covey et al in two thousand and seven also used the large SDSS dataset to establish a spectral typing pipeline based on flux-calibrated spectra from Pickles and derived spectral type versus SDSS color relations. This pipeline is known as “The Hammer” and was later utilized by West et al in 2011 and Lu et al in 20-19 to classify a great number of M dwarfs. A noteworthy recent effort on nearby northern M dwarfs that synthesizes earlier results and provides new types is that by Alonso-Floriano et al in 20-15, in preparation for the Calar Alto high-Resolution search for M dwarfs with Exoearths with Near-infrared and optical Echelle Spectrographs, CARMENES, survey.
Figure 2
(A) M dwarfs classified with types M0 to M9 dwarf by West et al in 2011 are shown on an empirical Hertzsprung Russell diagram, color coded by each of the ten full subtypes. note the sub dwarfs below the main sequence. Ten squares along each axis represent mean MG magnitudes and G to RP colors from Gaia Data Release 3 for these types, with error bars showing the standard deviations at each type. Embedded in the main sequence are standard stars for RECONS (circles) and values from Pecaut and Mamajek in 2013 stars, with projections for each shown along the G to RP axis.
(B) Spectra with resolution of a few angstroms for ten RECONS standard stars. Key spectral features used to establish the sequence are labeled at the top, where prominent telluric absorption regions are noted as gray bars. The color scheme is the same for both plots. The data shown here are available in the Supplemental Material.
Abbreviation: RECONS, REsearch Consortium On Nearby Stars.
Figure 2a shows M dwarfs from SDSS classified by West et al in 2011, who assigned types using The Hammer, and points are color coded by each full spectral subtype. Ten points along each axis show the mean absolute magnitude and color values for each subtype, with error bars representing the standard deviation ranges. Also shown are circular points representing RECONS spectral types and stars representing types from Pecaut and Mamajek in 2013. Overall, the spectral type trends are in agreement among all three systems. The Hammer program yields evenly spaced subtypes, whereas the RECONS and Pecaut sets include specific stars that can be used as standards, so the separations in MG in particular between specific subtypes vary somewhat, with relatively large gaps on either side of type M 4 dwarf, where the main sequence is the widest.
Metallicity, M over H: the star’s total metal abundance relative to the Sun, where log M over H equals 0 is defined as solar metallicity
The RECONS set traces the center of the main sequence and spans a relatively more restricted range in color than the other two sets, whereas the early-type stars in the Pecaut sequence are below the midpoint of the distribution, and the late-type stars are somewhat elevated.
As is evident in Figure 2a, assigned spectral types may differ by two full types for some M dwarfs for a given MG or G to RP value. In the era of Gaia, with its high-quality parallaxes and photometry that yield accurate absolute magnitudes, using MG is arguably better than using spectral types because M dwarfs within hundreds of parsecs can now be precisely placed on the HRD within 100 parsecs reddening effects are minimal, and MG values can be used to select potential M dwarfs even without the spectroscopic determination of types. At least within this horizon, the time-consuming process of securing spectra may not be needed for many research applications, and in fact, MG values offer higher fidelity in discerning general types of M dwarfs, given the errors in spectral subtypes. Of course, the subtleties of determining youth H alpha, rotation rates v sin eye, and metallicities M over H can still be explored effectively with spectra, and securing series of spectroscopic measurements remains critical for uncovering companions in radial velocity studies.
Section Three point three. Spectral Classification: M Sub dwarfs.
Because of their slow evolution, in addition to main sequence M dwarfs there is a set of cool, sub luminous M stars that lie below the main sequence that were called “sub dwarfs” by Kuiper in 19-39, a name that is analogous to subgiants for evolved stars. The first three M type sub dwarfs, which have absolute magnitudes 2 to 3 mag less luminous than main sequence stars of the same color, were reported by Kuiper in 19-40. The “sd” prefix for these sub dwarfs was used by Joy in 19-47 based on the Mount Wilson spectral classification system.
The introduction of the “sd” prefix began causing confusion in the late nineteen forties because “sub dwarfs” also began to be used for a class of under luminous blue stars, meaning types sdO and sdB. Unlike the old, low-metallicity M sub dwarfs, these hot sub dwarfs have broad Balmer absorption features or strong Helium two lines at 4,686 Angstroms, and their locations on the HRD are the product of binary star evolution, with Helium fusing in their cores. Thus, we are left with the unfortunate situation in which there are two entirely different classes of stars called “sub dwarfs”, a hot class that is the result of binary star evolution and a cool set of unevolved single stars that are among the oldest Galactic fossils.
The “sd” prefix continues to be used sometimes as part of the spectral type to indicate a cool sub dwarf, even expanding to sdM, esdM, or usdM to correlate with everlower metallicities. Instead, Roman in 19-55 and Jao et al in two thousand and eight have reasoned that for types later than G0, the spectral notation “VI” should be used for stars that are around 1 to 2 mag less luminous than their main sequence counterparts, thereby creating an appropriate luminosity class that separates sub dwarfs from main sequence dwarfs.
To identify M sub dwarfs spectroscopically, metallic hydride bands such as Magnesium Hydride, Calcium-Hydride, Aluminum-Hydride, or Iron-Hydride can be used because they are sensitive to metallicity. Eggen and Greenstein, 19-67, Boeshaar 19-76, Wing et al in 19-76, and Bessell in 19-82 suggested using metallic hydride band strengths to indicate luminosities and T-I-O band strengths as temperature indicators. After sub dwarfs were identified for a few decades, Gizis in 19-97 established an M sub dwarf classification system based on the metallic hydride bands of Ca-H one, Ca-H two, Ca-H three, and T-I-O Five, marked in Figure 2, although all stars shown are main sequence dwarfs. Lepine et al in two thousand and seven used a set of more than 400 cool sub dwarfs to refine the classification scheme, and a decade later Zhang et al in 20-19 proposed using an additional feature, Ca-OH at 6,230 to 6,240 Angstrom, to assist in characterization. Meanwhile, Jao et al in two thousand and eight proposed a spectral classification system that connected atmospheric models to empirical spectra and demonstrated both gravity and metallicity trends on the HRD for sub dwarfs.
Mass luminosity relations (MLRs): used to relate intrinsic bright nesses of stars to their masses, often using absolute magnitude measurements as proxies for luminosities.
A few comments related to L sub dwarfs are warranted here because a few of these objects may, in fact, be dwarf stars. Lepine et al in two thousand and three first identified LSR J 16 etc to be an L-type sub dwarf because it has weak bands of T-I-O and no detectable V-O. This star’s classification was later revised to be a peculiar sdM6, and it was subsequently found to be an astrometric binary.
A late L-type sub dwarf having strong metallic hydride bands and weak T-I-O bands, 2MASS J0 532 etc, was reported around the same time by Burgasser et al in two thousand and three, but it is a brown dwarf. Since then, some stellar L-type sub dwarfs with MG less than 17.8 have been discovered, with type sdL0 at 11.0 parsec, 340 Peta Meters such objects constitute a small population of the very oldest low-mass stars. Additional details about classifying L sub dwarfs are discussed by Kirkpatrick et al in 19-99, and others.
Section Three point Four. Masses.
Mass is the single most fundamental characteristic of a main sequence star because it determines its fuel-burning capabilities and structure. Composition, rotation, and magnetic properties, all of which change via evolution as stars age, are secondary attributes that affect how a star presents itself, but mass remains key to a star’s character throughout its life. Given that no M dwarfs have evolved in any meaningful way in the history of the Universe, mass is the primary driver of their fundamental observables, including their temperatures and consequent colors and spectral energy distributions, as well as their luminosities and absolute magnitudes. Although critical to stellar characterization, mass determinations remain one of the most difficult of stellar attributes to pin down, given that the gold standard of model-independent mass measurements remains orbital mapping of binary systems that takes considerable patience and endurance. One technique that has proven to be useful for more massive stars is astero seismology, but M dwarfs do not reveal detectable stellar oscillations useful for such measurements.
Nonetheless, we have made progress in determining red dwarf masses, which we now know span a range from 0.61 Solar Masses for the hottest types to 0.075 Solar Masses for the coolest stars. Popper’s (19-80) classic review of stellar masses included only four M dwarfs in two eclipsing systems, but the review by Liebert and Probst (19-87) provided a much richer set of 32 stars with masses of 0.07 to 0.50 Solar Masses. The first established mass luminosity relations, MLR’s for M dwarfs in the VJHK filters were presented within the relations spanning 0.08 to 2.0 Solar Masses by Henry and McCarthy, 19-93, where 26 stars with high-quality, at the time, masses less than 0.6 Solar Masses were included. Refinements to the V relation were made by Henry et al in 19-99 using measurements from the fine guidance sensors, FGS’s aboard the Hubble Space Telescope and expanded slightly to 33 M dwarfs by Delfosse et al in two thousand, who noted that a range of metallicities caused larger scatter in V relations than in the near-IR. Very high-accuracy masses with errors of less than 4 percent for 47 M dwarfs with masses 0.08 to 0.62 Solar Masses enabled by HST FGS measurements over more than a decade allowed Benedict et al in 2016 to provide more extensive MLR’s at V and K, and that V MLR remains the best available today. Dupuy and Liu in 2017 extended the mass measurements through the stellar sub stellar break with 38 masses of 30 to 115 Jupiter Masses, which were combined with previous efforts and updates to create a list of 62 binaries with mass sums by Mann et al in 20-19, from which a new MLR in K was derived. Overall, these efforts together result in MLR’s at V and K that can be used to estimate masses of M dwarfs to a few percent on average, although caution must be exercised for individual stars because other stellar attributes will affect the magnitudes observed.
For example, note the width of the HRD in Figure 1 illustrating that a single absolute magnitude in fact spans a range of color, with variations caused by characteristics other than mass.
The near future holds great promise for an expansive view of M dwarf masses because of the thousands of systems that will soon be available for mass determinations via the precise astrometry from Gaia.
Already, high astrometric errors in Gaia have been shown by Vrijmoet et al in 20-20 to be reliable indicators of multiplicity. Vrijmoet et al in 20-22 exploited this result, supplemented by results from long-term astrometry from the RECONS program and the literature, to resolve 211 M dwarf pairs using optical speckle imaging and present an initial set of five new high-quality orbits. The combination of forthcoming Gaia astrometric orbits for unresolved M dwarf binaries and high-resolution imaging will yield abundant opportunities for mass determinations and MLRs at V, I, where in fact, low-mass M dwarfs emit most of their flux, and K that are so refined that gradations due to metallicities, and perhaps rotation and magnetic properties, may be teased out.
With even better MLRs in hand, the M dwarf masses needed to derive orbiting exoplanet masses will become more reliable, and the characters of those worlds will be better defined.
A reliable relation for low-metallicity M sub dwarfs has remained elusive, but shifts in MLRs for these stars are presumably large. As shown by Baraffe et al in 19-98, theoretical work implies that MV for a 0.4 Solar Mass dwarf could differ by 1.0 mag between stars with metallicity 0 and minus 0.5, where the lower-metallicity dwarf is brighter. This offset is smaller in the K band, so targeted infraredspeckle and adaptive optics (AO) work could provide a more straightforward MLR for sub dwarfs at near-infrared wavelengths. Alas, similar to the challenges in studying cool sub dwarf multiplicity, Section 4.2, very few cool sub dwarf binaries have been identified that can be used to establish an empirical MLR, so rigorous tests of models at low metallicities remain to be done.
Section three point five. Radii.
M dwarf fundamental parameters also include radii, metallicities, and effective temperatures. Measurements of these parameters have been improved in the past few decades, but unlike measuring masses, derivations of these three parameters are intertwined and require additional assumptions beyond the simplicity of Kepler’s Third Law for masses. Accurate radii have become critical because they are needed to determine transiting exoplanet sizes, so there is significant motivation to get the host stars’ radii right.
The most common method used to “directly” measure the sizes of red dwarfs, which are no larger than about 0.6 Solar Radii, is to observe eclipsing binaries. These doubles may have an M dwarf as a companion to an FGK primary, two M dwarf components, or an M dwarf with a sub stellar companion. One nearby benchmark M dwarf ecliping binary is CM Draconis, GJ 630.1 AB, M 4.5 dwarf plus M 4.5 dwarf, 14.9 parsecs, 460 Peta-meters, a double-lined spectroscopic system with a white dwarf companion at 4.7 arc seconds. The near twin components orbit one another in 1.27 days, have radii of 0.25 and 0.24 Solar Radii, and are presumably in a tidally locked, synchronized spin, orbit resonance. This is a classic conundrum of eclipsing M dwarfs, to eclipse, the two small stars must be very closely separated, resulting in fast-orbiting, fast rotators that often exhibit frequent flares, Section 6.2, causing challenges for light curve modeling and, consequently, radii determinations. Additional complications include having to model spots and limb darkening; i.e., for M dwarfs there is no consensus on appropriate limb darkening corrections that depend on temperature, metallicity, and gravity. Nonetheless, even with these challenges, eclipsing M dwarfs often provide stellar radii reported to accuracies of a few percent.
The second direct method used to measure the radius of an individual star is long-baseline interferometry. Michelson and Pease in 19-21 pioneered this technique to measure the radius of Betelgeuse, a valuable description of the method is provided by Lawson. Interferometric baselines at the optical and IR wavelengths at which stars emit most of their flux have been extended to hundreds of meters, and stars as faint as M dwarfs can be reached by facilities such as the CHARA, Center for High Angular Resolution Astronomy, Array and the VLTI, Very Large Telescope Interferometer.
High-resolution efforts with these interferometers have now measured radii accurate to a few percent, with some observations made at near-IR wavelengths at which M dwarf fluxes are increased and limb darkening is less of a concern than at optical wavelengths, although resolution is sacrificed. Combining new measurements and literature values, Boyajian et al 20-12 presented radii of 0.14 to 0.57 Solar Radii for 16 M dwarfs of types M 0 dwarf to M 5 dwarf. Recently, Lachaume et al in 20-19 demonstrated an improved algorithm for measuring interference fringes, which could have a significant impact on both radii measurements and their uncertainties for stars smaller than the formal angular resolution limit of the interferometer. Thus, radii measurements may be improved, and uncertainties reduced, in the near future.
However, the M dwarf radii measured are larger than predicted by stellar evolutionary models by 10 to 15 percent at a given mass.
This discrepancy has been proposed to be the result of large-scale magnetic fields in these cool stars, but Feiden and Chaboyer in 20-14 showed that magnetic stellar models are unable to reproduce the properties of inflated, fully convective M dwarfs.
In addition, Kesseli et al in 20-19 have shown that neither fast rotation nor duplicity would inflate radii sufficiently to match the models for fully convective M dwarfs. Feiden and Chaboyer 20-12, also demonstrated that by revising standard stellar evolutionary models, the radius discrepancy can be reduced to 4 percent but point out that the uncertainties in empirical radii measurements may be too large to stress-test the models at this level. Another possible cause of the inflated radii in real stars compared to models could be the presence of starspots that could cause stars to appear larger by up to 10 percent, and cooler by a few hundred degrees Kelvin, at least for stars with large areal spot coverage. Along these lines, a recent study by Wanderley et al in 20-23 shows that models with spots could account for the inflated young M dwarfs in the Hyades, assuming the inflated stars have spots covering 20 to 40 percentof their surfaces.
Section Four. POPULATION ASPECTS.
Section Four point one. Population Based on the 10 Parsec Sample.
Arguably the single most important attribute of M dwarfs is their overwhelming population relative to other stars. Here, we base our understanding of the M dwarf contribution to the stellar population on the census of stars within 10 parsecs, for which the statistics are summarized in Table 2. Three out of every four stars, a full 75 percent in the Solar Neighborhood are red dwarfs, meaning that they not only constitute the most common type of star but offer by far the greatest number of options for planetary environments and life, see Section 7.
Table 2. Census of stars within 10 parsecs of the Sun.
Spectral types, and primaries and companions are listed in the text.
Lutz Kelker bias: a statistical effect caused by random parallax errors leading to the underestimation of stars’ distances.
Malmquist bias: an observational effect that leads to the preferential detection of intrinsically bright objects.
In fact, even though individually each star has a relatively thin realm in orbital semi major axis where temperatures are appropriate for liquid water on planetary surfaces, en masse they include over one-third of all potentially “habitable real estate”. Careful summing of total mass for each stellar spectral type also reveals that, although small, M dwarfs in fact contain more mass than any other type, thereby giving them together outsized gravitational prowess compared to other stars.
Although limited, the 10-parsec horizon is the distance to which M dwarfs have been effectively fully discovered and vetted, as both primaries and secondaries, through a combination of more than a century of ground-based parallax work and recent space-based missions. The 10-parsec sample has been specifically studied by Kuiper, 194-2, the RECONS group as detailed by Henry et al in two thousand and six, 20-18 and updates at recons dot org, and recently by Reyl eet al, 20-21. It is important to note that a simple trawl of GDR3 results will not yield accurate population statistics, of the 269 stellar systems within 10 parsec, 22 (8 percent) do not have astrometric solutions for any of their components in GDR3, including five very bright stars as well as 17 close multiples that contain 36 red dwarf components. So, a GDR3 trawl alone would result in an undercount of red dwarfs by 13 percent, and the shortfall would be even worse if many of the unresolved red dwarf companions were not uncovered through scrutiny of each higher-mass system.
Ground-based progress through the twentieth century in revealing the population of nearby M dwarfs via their trigonometric parallaxes can be tracked using the series of Yale Parallax Catalogues (YPCs), the fourth edition of which was published by van Altena and colleagues in 19-95, see the electronic version in van Altena et al from two thousand and one. Significant contributors of M dwarf parallaxes in the twentieth century, defined as those providing at least dozens of high-quality measurements, include efforts from the Allegheny Observatory, led by Gatewood), the McCormick Observatory (Ianna), the Sproul Observatory (Heintz), the United States Naval Observatory (Dahn, Monet) the Van Vleck Observatory (Upgren, Weis), and Yale Observatory (van Altena). By the time of the final YPC edition, 122 (63 percent of the 194 M dwarf systems within 10 parsec had been identified. The final YPC was published before any results were forthcoming from the Hipparcos mission, yet additional ground-based efforts after Hipparcos contributed many first parallaxes for M dwarfs beyond the spacecraft’s faint limit. The largest efforts were those by the RECONS group (Henry, Jao), the Carnegie group (Boss, Weinberger), and the M Earth group (Charbonneau), as well as continuing efforts at the United States Naval Observatory. After the YPC came the space-based augmentation of the 10-parsec sample by Hipparcos, the first all-sky astrometry mission that was launched in 19-89, which added 18 new M dwarf systems. Yet, the faintest new systems found by Hipparcos had V equals 12.2, leaving many fainter red dwarfs to be discovered. The largest contributor to the population of 10 parsec M dwarfs post-Hipparcos is the RECONS team, with 36 new systems, whereas all other groups contributed a total of 16 systems.
The combination of successful ground-based efforts and the Hipparcos sky sweep did not leave (m)any new M dwarf systems to be revealed for the first time by Gaia, as it contributed only two new systems to the 10 parsec sample, both of which had previous parallaxes placing them just beyond 10 parsec. The conclusion is that there remain very few, if any, new stars to be discovered within 10 parsec, meaning that the sample is effectively volume-complete, thus, the census numbers presented in Table 2 are secure. Because of the high precisions of parallaxes and photometry now possible from ground and space, the Lutz Kelker bias and the Malmquist bias are not relevant for the 10 parsec sample, for which the median parallax error is only 0.03 milli-arc-seconds, and for which targets have photometric measurements better than a few percent. Predictably, there are no rare O and B stars within 10 parsec of the Sun, only modest numbers of solar-type FGK stars, and M dwarfs dominate the population.
Figure 3 illustrates the population schematically in a convenient form, where 100 dwarfs are used to represent the Solar Neighborhood population, and the counts within those 100 representative stars are shown.
Figure 3. A schematic representation of stellar populations in the Solar Neighborhood, illustrating that M dwarfs account for three of every four stars. The full census numbers for stellar types within 10 parsecs are given in Table 2. There are no O or B stars so close.
The 10-parsec sample offers an initial glance at one of the most difficult of all characteristics to determine for M dwarfs, their ages. Because they are so long-lived, red dwarfs present extreme ages that set them apart from all other types of stars, yet their ages are difficult to discern because the stars remain relatively unchanged since birth. There are two very young and one very old red dwarf systems even within 10 parsecs of the Sun. The nearest young system is “A-P” Col at 8.4 parsec, 260 Peta meters, with an age of around 40 Mega-years. Found just within the horizon at 9.8 parsecs, 302 Peta meters, the second young system is A-U, A-T Mic, a triple set of M dwarfs that is a member of the Beta Pictoris group with an age of about 20 Mega-years. A-U Mic is known to be surrounded by a disk and has up to four reported planets. The binary A-T Mic is around 1.3 degrees from the primary on the sky, about 50,000 Astronomical Units, and the two close components orbit one another in approximately 150 years.
At the other age extreme, Kapteyn’s Star is the nearest representative of a rare, cool sub dwarf close to the Sun, at a distance of only 3.9 parsec, 120 Peta Meters. With an age of perhaps 10 Giga-years or more, it is an ancient, sub luminous red dwarf likely from the Galactic halo that is currently moving quickly through the Solar Neighborhood, it has the second largest proper motion, 8.7 arc seconds per year, of any star, after only that of Barnard’s Star, 10.4 arc seconds per year. A likely second sub dwarf within 10 parsec is C-F U-M-a, also known as Groombridge 18-30, although its spectral type is early K rather than M. Given two very young and two very old systems within 10 parsec, the rate for each age extreme is about 1 percent of all systems. Alas, because it is difficult to pin down the ages of M dwarfs, we also do not know the ages of any planets they host.
Nonetheless, the population of long lived red dwarfs provides the best opportunity for understanding the entire history of the Milky Way because their ages stretch from only a few percent of the age of our Galaxy to fossils that have stood the test of time and have had the full dynamic experience throughout the Milky Way’s history.
Section Four point two. Multiplicity.
Whether or not any star is solitary or is orbited by other stars, brown dwarfs, planets, or debris provides insight into its formation and evolution. For example, single stars and components in wide binaries are presumably better hosts of planetary systems because disks of material can create planets in stable orbits that are not wrecked by close-in stellar companions. For context, among the members of the 10-pc sample, the 194 M dwarf primaries break down into 141 singles, 41 doubles, 10 triples, 1 quadruple, and 1 quintuple, indicating that 27 percent of systems are multiple. Of the 53 M dwarf multiples within 10 parsec, 49 of the primaries have exclusively red dwarf companions and 4 have brown dwarf companions, implying that brown dwarfs form as companions to red dwarfs less than one-tenth as often as stars.
Many searches for stellar companions to M dwarfs have been carried out, from early IR imaging efforts by Skrutskie et al in 19-89, 55 stars, and IR speckle work by Henry, 19-91 74 stars, to the HST, NICMOS, Near Infrared Camera and Multi-Object Spectrometer, work of Dieterich et al 20-12, 126 stars, the lucky imaging surveys of Janson et al 20-14, 286 stars, and Cortes Contreras et al in 20-17, 490 stars, and the IR AO study of Ward-Duong et al, 20-15, 245 stars.
The most comprehensive assessment of M dwarf multiplicity to date is that by Winters et al, 20-19, who evaluated all types of M dwarf systems and their companions within 25 parsec and provided an overview of 16 previous surveys in their table 1, including all of the surveys just mentioned. Their all-sky examination of 1,120 systems with M dwarf primaries involved new surveys for companions with separations of 2 to 300 arc seconds, augmented with a comprehensive literature search, and yielded a multiplicity rate of 26.8 plus or minus 1.4 percent, which is identical to the 10-parsec fraction. With so many systems surveyed, it was found that there is a broad peak in the separation distribution of the companions at 4 to 20 Astronomical Units, mean on Solar System scales. Two known caveats reported by the authors are that more than 50 stars appear to have as-yet-unconfirmed close companions that would bump up the multiplicity rate and shift the most common separations to smaller orbit sizes, and that the sample targeted was far from complete, as it was created before Gaia results were available.
Future efforts should concentrate on expanded surveys and lower-mass companions, including brown dwarfs and planets of all types. Initial forays into this realm are underway, primarily as radial velocity surveys, for example by Delfosse et al in 19-99, 127 stars, and Reiners et al in 20-18, 324 stars, and speckle imaging studies by Vrijmoet et al in 20-22, 333 stars, but the full suite of companions is unlikely to be well understood until a few thousand M dwarfs have been surveyed comprehensively.
To this end, the RECONS group is constructing a fully vetted sample of M dwarf systems out to 25 parsecs, which includes over 3,000 primaries. With such a sample in hand, many groups can combine efforts to discover and explore the myriad companions with masses from 0.6 Solar masses down to terrestrial planets, a factor of a million in mass, with goals of ultimately understanding the star and planet-formation processes for all types of M dwarfs.
The multiplicity assessments described above include M dwarfs in field samples and provide clues about the distributions of mass ratios and orbit types, Section 4.3. Additional studies that reach further afield address M dwarf multiplicity for younger and older stellar populations.
Bergfors et al in 20-10, surveyed 124 active, presumably young, M dwarfs and found 44, 35 percent to have companions, whereas Janson et al in 20-12, expanded on that work and observed 337 similarly active M dwarfs, finding 85, 25 percent to be multiple. Because the stars are rather more distant than the Solar Neighborhood sample of Winters et al in 20-19 and not all separations were included, these results imply that young M dwarfs may have companions somewhat more often than older field samples.
At the other end of the age spectrum, studying the multiplicity of M-type halo sub dwarfs yields clues about the binary-formation history for older populations. The challenges of studying sub dwarfs are their intrinsic low luminosities compared to their main sequence counterparts and far lower space densities, forcing surveyors to reach to more distant objects and exacerbating the faint target problem. Since the year two thousand, several small and modest surveys have been conducted for cool sub dwarf companions. Initial surveys include those by Gizis and Reid in two thousand, who used HST, WFPC2, Wide Field Planetary Camera 2, to observe 11 cool sub dwarfs, none of which were resolved; Lepine et al in two thousand and seven, who resolved 1 of 18 M sub dwarfs using the AO system at the Lick Observatory, and Riaz et al ion two thousand and eight, who reported one visual binary using HST, ACS, Advanced Camera for Surveys, among 19 M sub dwarfs. Jao et al, in two thousand and nine observed a bigger sample of 62 confirmed K and M sub dwarfs within 60 parsecs using optical speckle techniques and determined a multiplicity rate of 26 plus or minus 6 percent. Ziegler et al in 20-15 again increased the size of the sample by selecting 344 sub dwarf candidates from a reduced proper motion diagram and resolved 12.5 plus or minus 1.9 percent of them using the Robo-AO system. Recently, González-Payo et al, 20-21 used Gaia results to create a list of 214 M and L sub dwarfs and found a multiplicity rate of only 1 plus 2.0 minus 1.0 percent for projected separations of up to more than 700,000 Astronomical Units using the GDR3 catalog. However, this sample will necessarily miss most close companions, so the rate is quite low, as might be expected given that many M dwarf companions are within a few tens of astronomical units. Although the multiplicity rate remains somewhat unclear for sub dwarfs because results vary depending on how the samples are selected and the detection methods, the rate is perhaps lower for M sub dwarfs than for field samples, pointing to a likely decreasing multiplicity rate for M dwarfs from young to old stars. Two explanations, both of which may potentially be at play, are that lower metallicity sub dwarfs simply do not form companions as they might in higher-metallicity environments and, or multiples are destroyed over time.
Section four point three. Orbits.
One of the research areas that is advancing quickly at present is the assessment of M dwarf orbits, and a windfall of new orbits is expected in the next few years via future Gaia data releases.
The first evaluation of M dwarf orbital configurations carried out using the classic eccentricity (e) versus orbital period (P orb) plot, which effectively maps orbital shapes and sizes, was presented by Udry et al in two thousand, who characterized 48 red dwarf binaries using radial velocity observations.
The tidal circularization period appeared to occur around 10 days, and there was a hint of structure in the distribution, implying a lack of circular orbits at longer periods, although the data were sparse. Recent work by Vrijmoet in 20-23 has expanded the sample to 193 orbits for M dwarf multiples with periods of less than a day to 30 years, corresponding to orbital semi major axes less than 10 Astronomical Units. Vrijmoet finds that the orbital circularization period for M dwarfs is around 7 days, which is much shorter than the around 12-day period for solar-type stars. It also appears that there are very few systems with P orb equals 5 to 30 years in circular orbits. The few systems with e less than 0.1 all have at least one component of very low mass, suggesting that their early dynamical evolution is dominated by migration through circumstellar disks, whereas more massive systems do not end up in nearly circular orbits.
As discussed in the work of Winters et al in 20-19, which targeted 310 M dwarf pairs, many of which were too wide to have orbits, and additional closer systems studied by Vrijmoet, 20-23, the mass ratios of M dwarfs in multiple systems point to equal mass systems being the most common, meaning mass ratios q around 1. This is particularly true for the close systems. The distribution for q equals 0.5 to 0.9 is relatively flat for both samples, and then for mass-discordant systems with q less than 0.5 there is a tail off to lower masses and virtually no systems with q less than 0.1. A caveat noted by both sets of authors is, however, that the high-mass ratio systems are the most difficult to detect in both imaging and radial velocity surveys, although in the latter case the efforts by Baroch et al in 20-18 and Winters et al in 20-20 have recently added important spectroscopic binaries via sensitive searches.
Thus, a key area of future work is to target a volume-complete sample of M dwarfs to prevent biases and to reach as far down in mass for companions as is feasible to reveal the true natures of the mass ratios between these stars and their companions.
Section four point four. Red Dwarf Luminosity and Mass Functions.
Here, we evaluate the red dwarf members of the population relative to one another in the forms of their luminosity function (LF) and mass function (MF), both of which illustrate the extreme differences between the largest and smallest types of these small stars. The LF at optical wavelengths has been known to turn over for nearly a century, Luyten in 19-38, and in 19-68, Reid et al in 19-95, Gaia Collaboration 20-21, although shifts occur in the precise peak locations owing to the choice of filter band pass, the parallax sets available at various times, and treatments of unresolved multiples. As discussed above, mass is the most critical aspect of a star, so the MF is therefore the more important snapshot of the diversity of the M dwarf population.
Work on the MF for red dwarfs has led to conflicting interpretations: Scalo, in 19-86, Reid in 19-87, and Bochanski et al in 20-10 concluded that the MF turned over, typically reaching a peak near 0.2 to 0.3 Solar Masses, whereas Dantona and Mazzitelli in 19-86, Henry in 19-91, and Winters et al in 20-19 contended that the MF increases down to at least 0.1 Solar mass. Particularly revealing is that the latter study reached out to 25 parsecs to include more than 1,000 M dwarfs before Gaia results were available, and even though the sample was known to be incomplete, the MF was clearly rising to the lowest masses.
As discussed in Section 4 point 1, the modern 10-parsec sample is ideal for deriving the most accurate stellar population statistics because the 269 systems of all spectral types within 10 parsec have been completely searched and vetted for at least companion stars in long-term astrometry, radial velocity, AO, and speckle imaging surveys. However, the 280 M dwarfs within 10 parsec constitute a relatively small population. In order to bolster the statistics for LF and MF, here we reach a bit further and focus on red dwarfs with parallaxes of at least 60 mas, creating a list of nearly 1,000 M dwarf systems, by Madison LeBlanc.
Gaia provides key additions to this more distant horizon of 16.7 parsecs, where G equals 18.9 for stars at the end of the main sequence at MG equals 17.8, resulting in a sample that is comfortably brighter than Gaia’s detection limits. The sample has been constructed using GDR3 20-23 and supplemented with additional systems with parallaxes from other sources, but as presented here includes only systems with red dwarf primaries. As pointed out by Henry and McCarthy in 19-90 and reaffirmed by Winters et al in 20-19, de blending multiples is critical when determining accurate LF and MF distributions, so we provide the results for a de blended 16.7 parsec sample to the extent that it can be done. This is largely possible for systems in which the M dwarf is a primary, but systems with more massive primaries oft
-
12:19
T-SPLY
6 hours agoDemocrats Start Economic War With El Salvador!
12K12 -
13:42
SantaSurfing
4 hours ago4/24/2025 - Part 1 on Endless Frontiers Efficient & Powerful Tesla Homes! Revolutionary!
16.5K19 -
UPCOMING
VOPUSARADIO
7 hours agoPOLITI-SHOCK! "Destroying The Narrative"! W/ 3 Special Guests
23 -
58:12
Donald Trump Jr.
4 hours agoInterview with CIA Deputy Director, Plus FBI's Major Maduro-TDA Discovery | Triggered Ep236
101K113 -
58:56
BonginoReport
2 hours ago“Womanosphere” Podcasts Trigger Leftist Media - Nightly Scroll w/ Hayley Caronia (Ep.34) - 04/24/25
36.4K28 -
42:35
Dad Dojo Podcast
6 hours ago $1.17 earnedEP29: Lebron Turns Heel, Steroids and more
15.4K2 -
1:16:21
Kim Iversen
4 hours agoXi and Zelensky IGNORE Trump: Is America's Power Crumbling?
57.8K54 -
LIVE
TheTapeLibrary
10 hours ago $0.24 earnedThe Disturbing Case of the Becker Haunting (FULL PARANORMAL DOCUMENTARY)
151 watching -
LIVE
Quite Frankly
5 hours ago"Op Sea Spray, Assassination Files, MK-Internet" ft. Eric Hunley 4/24/25
872 watching -
DVR
LFA TV
8 hours agoAmerica’s Shameful Foreign Policy | TRUMEPT DAILY 4.24.25 7PM
7.49K