Premium Only Content
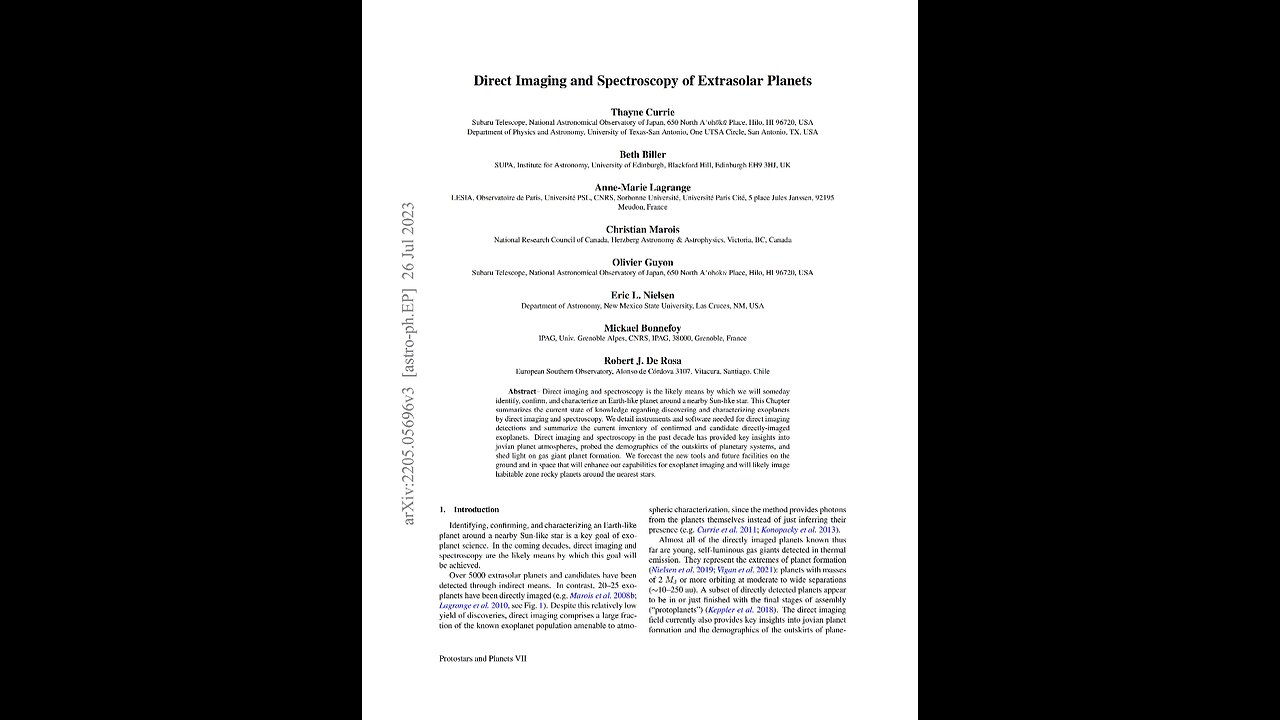
Direct Imaging of Extrasolar Planets. Thayne Currie,2023. A Puke (TM) AudioPaper
arXiv:2205.05696v3 [astro-ph.EP] 26 Jul 2023
https://arxiv.org/abs/2205.05696
https://arxiv.org/pdf/2205.05696.pdf
https://rumble.com/v3t4yzj-index-of-science.-music-by-dan-vasc.html
Direct Imaging and Spectroscopy of Extrasolar Planets.
Thayne Currie, and others, 2023.
Abstract. Direct imaging and spectroscopy is the likely means by which we will someday identify, confirm, and characterize an Earth-like planet around a nearby Sun-like star. This Chapter summarizes the current state of knowledge regarding discovering and characterizing exoplanets by direct imaging and spectroscopy. We detail instruments and software needed for direct imaging detections and summarize the current inventory of confirmed and candidate directly-imaged exoplanets. Direct imaging and spectroscopy in the past decade has provided key insights into Jovian planet atmospheres, probed the demographics of the outskirts of planetary systems, and shed light on gas giant planet formation. We forecast the new tools and future facilities on the ground and in space that will enhance our capabilities for exoplanet imaging and will likely image habitable zone rocky planets around the nearest stars.
1. Introduction.
Identifying, confirming, and characterizing an Earth-like planet around a nearby Sun-like star is a key goal of exoplanet science. In the coming decades, direct imaging and spectroscopy are the likely means by which this goal will be achieved.
Over 5000 extrasolar planets and candidates have been detected through indirect means. In contrast, 20 to 25 exoplanets have been directly imaged, see Figure one. Despite this relatively low yield of discoveries, direct imaging comprises a large fraction of the known exoplanet population amenable to atmospheric characterization, since the method provides photons from the planets themselves instead of just inferring their Presence.
Almost all of the directly imaged planets known thus far are young, self-luminous gas giants detected in thermal emission. They represent the extremes of planet formation, planets with masses of 2 Jupiter Masses or more orbiting at moderate to wide separations, around ten to 250 astronomical units.
A subset of directly detected planets appear to be in or just finished with the final stages of assembly, protoplanets.
The direct imaging field currently also provides key insights into Jovian planet formation and the demographics of the outskirts of planetary systems.
Remarkably, the direct imaging method enables the acquisition of hundreds to thousands of spectroscopic data points on exoplanets in a few hours of telescope time. This wealth of information can critically constrain individual planet atmospheric properties, for example temperature, clouds, chemistry, and gravity, as well as the atmospheric evolution of gas giants as a population.
The first direct images and spectra of planet candidates relied on facility, general-use adaptive optics (AO) systems to deblur starlight or space telescopes, meaning the Hubble Space Telescope, coupled with simple coronagraphs. The past decade has seen the development, demonstration, and honing of dedicated extreme AO systems coupled with advanced coronagraphs and sophisticated post-processing methods, allowing the detection of planets that are fainter and less massive and, or located at smaller angular separations that probe tighter orbits.
In-development extreme AO systems and upgrades to first-generation extreme AO systems will provide images of many exoplanets near the ice line.
The Coronagraphic Instrument on NASA’s Roman Space Telescope (Roman-CGI) could provide the first detections of mature planets in reflected light, Figure Two.
Planned ground-based extremely large telescopes and proposed space missions promise to make the discovery and confirmation of a habitable, Earth-like exoplanet around a Sun-like star a reality within the next 25 years. These facilities will endeavor to reveal biomarkers, for example water, oxygen, ozone, in individual systems. Their surveys will provide the first assessment of potential habitability around stars of different masses and thus the true context for life on Earth. Technological innovation, atmospheric characterization, and demographic studies of young Jovian planets over the past decade provide first, key steps towards this goal.
In this Chapter, we provide an updated description of the state of our knowledge about detecting and characterizing exoplanets by direct imaging. The last dedicated direct imaging review chapter in Protostars and Planets was written in 2007, before the first incontrovertible exoplanet imaging detections: super Jovian planets around HR 8799 and Beta Pictoris.
Previous reviews from Traub and Oppenheimer in 2010 and Bowler in 2016 bracket the start and end of the era of direct imaging surveys with facility, conventional AO systems. Now, more than 5 years later, the first generation of extreme AO surveys have ended, bringing new discoveries, fundamentally new insights into atmospheric properties of individual systems and imaged planet demographics, motivating substantially more powerful instrumentation and tangible plans for directly detecting true solar system analogues, including Earths.
This Chapter is pedagogical in nature and is organized as follows. In Section 2, we outline key direct imaging instrumentation and methods, describing the challenges in achieving planet detections by direct imaging, and detailing critical, novel hardware and software needed to image planets.
Section 3 summarizes our current inventory of directly imaged exoplanets, discusses challenges with interpreting direct imaging detections, and outlines synergies with other detection techniques. Section 4 focuses on atmospheric characterization of exoplanets by direct imaging and spectroscopy, using other substellar objects, meaning brown dwarfs, as anchors to provide empirical constraints on exoplanet atmospheres and employing atmospheric models to infer intrinsic properties, including clouds, chemistry, and gravity.
Next we overview the architecture of directly imaged planetary systems gleaned from astrometric monitoring, dynamical models, and planet-disk interactions, Section 5.
Section 6 summarizes recent direct imaging surveys, the occurrence rates and demographics derived from them, and what these results mean for models of Jovian planet formation.
Finally, in Section 7 we forecast the future of direct imaging, describing how technological innovations and new, vastly more powerful facilities may provide humanity with a glimpse of a habitable world for the first time.
2. Direct Imaging Instrumentation and Methods.
Direct imaging detections require separating the halo of bright, highly structured scattered starlight from faint exoplanet light. The key metric used to determine the detectability of extrasolar planets is the planet-to-star contrast ratio at a given off-axis angular separation: the contrast ratio needed for a detection varies with planet properties.
Section 2.1.
Critical hardware like AO, or, more generally, wavefront control systems consisting of sensors and deformable mirrors, and coronagraphs sharpen and then suppress scattered starlight, Section 2.2 to achieve deep raw contrasts.
Novel observing techniques allow post-processing algorithms to further remove residual starlight, increasing achievable contrast ratios and thus improving planet detection capabilities, section 2.3.
2.1. Detectability of Planets by Direct Imaging.
Even in the absence of an atmosphere to blur starlight, an astronomical image of a point source, for example a distant star, will not be a single point: its intensity distribution follows from the Fourier transform of the telescope pupil function.
For a simple unobscured circular aperture, the image intensity follows an Airy pattern whose values compared to the peak intensity I zero at angular separation rho, effective telescope aperture diameter D, and wavenumber k equals two pi over lambda is the standard airy pater using a Bessel function J1, where J1 is the Bessel function of the first kind of order one. The first null of the Airy function occurs at about 1.22 lambda over D. The full-width at half maximum (FWHM) of this point source on the sky, defining the telescope diffraction limit, is given by equation one:
Theta in arc seconds is about zero point two one times wavelength in microns over telescope diameter in meters.
Even current telescopes in principal have the angular resolution needed to image planets on solar system-like scales orbiting nearby stars. An Earth twin orbiting at a projected separation of 150 Giga-meters, or 1 A-U from a Sun-like star at 308 Peta-meters, or 10 parsec subtends an angle of a tenth of a second of arc, or a half a micro-radian.
Diffraction limited imaging with the Hubble Space Telescope at optical wavelengths or with 8 to 10 meter class telescopes like VLT, Subaru, and Keck at near infrared (IR) wavelengths is sufficient to resolve objects at Earth-Sun projected physical separations out to a distance of three to six hundred Peta-meters, which is 10 to 20 parsec. However, the star’s halo light must be reduced through hardware and software to a level sufficient to make the planet’s light detectable. The starlight suppression level required to detect a planet by direct imaging depends on whether the detection is in reflected light or thermal emission and varies with the planet angular separation, age, size, temperature, and other properties, see Figure two.
2.1.1. Reflected Light.
Planets reflect the light of the stars they orbit. At optical wavelengths, the contrast ratio for a planet in reflected light can be approximated as equation two:
Contrast Ratio is a function of square of the ratio of planet radius to planet-star separation times an Albedo function, and a phase function.
The Albedo and the phase function depend on the planet’s atmospheric properties.
For a Lambertian phase function, valid for high albedo atmospheres, the phase function follows a simple trigonometric relation.
Other potential scattering phase functions include isotropic scattering and Rayleigh scattering.
The value of the phase function is considerably lower for Rayleigh scattering appropriate for Jovian atmospheres at angles of sixty to ninety degrees.
For an exo-Jupiter and exo-Earth emitting as Lambertian spheres with measured geometric albedos of 0.52 and 0.367, these contrasts at maximum elongation reduce to equations three and four in the text.
Contrast ratios are of the order of ten to the minus nine to ten.
At V band, a Jupiter and Earth at around 300 Peta-meters, 10 parsec, will then have apparent magnitudes of slightly greater than 27 and 29, respectively, and require starlight removal at angular separations of one half and one tenth arc second better than one part in ten to the minus nine and ten to the minus ten, respectively. These required contrasts are well beyond the capabilities of current ground and space-based high-contrast imaging instruments. Thus far, exoplanet direct imaging has therefore focused on the detection of thermal emission from self-luminous Jovian planets.
2.1.2. Thermal Emission.
Like brown dwarfs, Jovian planets cool and contract with time, releasing gravitational potential energy as thermal emission. At ages of one to ten mega year, models for the luminosity evolution of one to ten mass of Jupiter exoplanets predict temperatures of around 500 to 3000 Kelvin and radii up of r p around 2.5 to 3 Radii of Jupiter. By 1 Giga year, these models predict that these planets cool to temperatures T less than around 500 Kelvin and contract to Jupiter-like radii, r p around one to one point two RJ.
Thus, thermal emission from young Jovian exoplanets peaks at near-to-mid IR wavelengths at the youngest ages, moving to well into the mid-IR at older ages, see Figure three.
The contrast ratio for planets in thermal emission depends on the radius and effective temperature of the planet and star and other properties, equation five.
Which can include a function of temperature and atmosphere composition, the thermal flux from the planet as a function of wavelength, The Flux function is the thermal flux from the star as a function of wavelength, and X depends on the planet’s atmospheric characteristics, such as clouds, chemistry, and gravity.
Figure three shows how the contrast ratios vary for various types of exoplanets orbiting a G2 dwarf star. In the near-IR, young super Jovian, ten mass Jupiter exoplanets similar to Beta Pictoris B and HR 8799 cde are roughly a factor of ten to the minus three to ten to the minus five fainter than a Sun-like star. In the red optical, predicted contrasts from these planets are 100 times larger; however, mid-IR contrasts are a factor of 10 smaller. Jovian exoplanets of similar masses around much older stars are far cooler, another factor of 100 to a 1000 fainter in the red optical and near-IR, but remain bright in the mid IR. A true Jupiter analogue emits negligible thermal emission for wavelengths greater than four to five microns. Rocky terrestrial planets also emit thermally.
An exo-Earth analogue with an effective temperature of 260 Kelvin has blackbody emission peaking at 10 microns, its contrast ratio is ten to the minus seven, a factor of 1000 shallower than its reflected light contrast.
2.2. Wavefront Control and Coronagraphy.
2.2.1. Atmospheric Wavefront Control for Direct Imaging.
Wavefront errors, whether induced by atmospheric turbulence on the ground or intrinsic to an optical imaging system on the ground or in space, substantially limit an image system’s achievable contrast. Left unmitigated, these errors preclude the direct detection of exoplanets. In the past decade, the direct imaging field has made key strides in wavefront control hardware and software to drastically reduce wavefront errors and has developed sophisticated coronagraph designs to further suppress diffracted starlight.
For ground-based imaging systems, turbulence arising from many atmospheric layers along the path from the star to the telescope induces changes in the optical path length difference of starlight, blurring images.
These aberrations must be corrected by an AO system consisting of wavefront sensors and deformable mirrors (DMs). An AO system splits incoming light from a guide star between the science detector and a wavefront sensor to measure and then correct, with a DM(s), atmospheric turbulence distorting the incoming wavefront, sharpening starlight at the image plane.
Key terms driving the wavefront error budget for an AO system include:
1) Measurement error sigma m, which depends on the noise properties of a wavefront sensor and the guide star brightness,
2) Temporal bandwidth error Sigma T, which depends on the AO system time lag tau compared to the atmospheric coherence time tau zero, and,
3) The fitting error sigma f which depends on the coherence length r zero compared to the DM actuator density. The quadrature-added sum of these terms sets the Strehl ratio, a measure of the optical quality of the image, and the raw contrast versus angular separation. At the angular separations relevant for most current planet searches theta around zero point two to one arc second, wavefront measurement error and temporal bandwidth error set the contrast floor. Wavefront chromaticity and non-common path aberrations due to instrument optics can also limit contrast.
Facility, conventional AO systems were used for the first direct imaging searches. They typically sample and correct the incoming wavefront at frequencies of p;oint two to one kilo Hertz and use DMs with a few hundred actuators: aberrations are measured by standard Shack-Hartmann wavefront sensors. These systems yielded partial corrections, achieving modest Strehl ratios of zero point one to zero point four at near-IR wavelengths focused on by most exoplanet imaging Searches.
The past 5 to 10 years have seen the deployment of numerous extreme AO systems on 5 to 10 meter telescopes, which have wavefront control loop speeds of greater than one kilo Hertz and DMs with more than a 1000 actuators. Examples of extreme AO systems include the Large Binocular Telescope Adaptive Optics system, LBTAO, PALM-3000 on the Hale telescope at Palomar Observatory, the Gemini Planet Imager on Gemini South, and the Spectro-Polarimetric High-contrast Exoplanet REsearch instrument (SPHERE) at the VLT, the Subaru Coronagraphic Extreme Adaptive Optics project (SCExAO) on the Subaru Telescope, and MagAO-X on the Clay telescope at the Magellan Observatory.
These systems obtain higher-quality AO corrections, Strehl ratios of pont seven to zero point nine five at 1.6 microns, and yield a factor of 10 to a 100 deeper contrast at sub-arc second separations than conventional AO systems.
Extreme AO systems have relied on hardware developments in three key areas to operate high-performance AO correction: detectors, deformable mirrors, and computing hardware. Most extreme AO platforms utilize fast ultra-low noise detectors like Electron-Multiplying Charge-Coupled Devices (EMCCDs) to record stellar photons used for wavefront sensing with reduced measurement errors.
High-actuator count DMs are now available. For example, the micro-electromechnical-type DMs (MEMS) offer unprecedented actuator density around 10 actuators per square millimeter, allowing for fairly compact extreme AO instruments. Other systems employ adaptive secondary mirrors (usually voice-coil DMs) whose low emissivity makes them especially well suited for exoplanet imaging in thermal IR. High performance computing hardware with low latency can handle the demanding computation requirements of fast, high order extreme AO systems.
Extreme AO systems also differ from conventional AO systems in their optical design and system architecture. Wavefront sensors (WFS) optimized for speed, sensitivity, accuracy and precision such as the Pyramid wavefront sensor have been adopted by many leading extreme AO systems (top panel of Figure four, while the more established Shack-Hartmann WFS approach has been upgraded with spatial filtering to improve performance. Wavefront correction is often performed in two steps: a conventional coarse “woofer” correction is followed by a faster and more accurate “tweeter” correction.
Extreme AO systems also include dedicated sensing and control of pointing for precise co-alignment of the star and coronagraph mask, which is essential to maintain high contrast, especially at the smallest angular separations.
Advances in wavefront control algorithms are also enabling significant improvements in high-contrast imaging performance. Recent advances include predictive control and sensor fusion. These and other new software, hardware advances are described in Section 7.1.
2.2.2. Optical Starlight Suppression.
Starlight suppression is primarily performed by dedicated coronagraph optics: phase and amplitude masks deployed in the beam to remove starlight while preserving planet light. These optical elements include occulters in the image plane to directly block out on-axis starlight, as well as optical elements in the pupil plane to manage the telescope diffraction pattern. Many coronagraph designs and approaches are available providing a wide range of performance characteristics. For example, the well-established conventional Lyot coronagraph combines an occulter in the image plane with a Lyot stop in the pupil plane to deliver robust performance at moderate contrast and large angular separations.
Phase-mask coronagraphs or interferometric designs, for example, the vector vortex coronagraph, can be highly optimized for deep contrast at small inner working angles, Figure four, bottom panel. The highest-performing coronagraphs are also the most demanding in terms of wavefront quality and stability. Consequently, extreme AO systems first adopted Lyot coronagraphs, while more recent systems or upgrades deploy higher performance solutions as the corresponding wavefront quality improves.
Wavefront control is also becoming an important component of starlight suppression in the form of speckle control. In speckle control, a feedback loop from the post-coronagraph image to an upstream deformable mirror allows for residual speckles to be measured and canceled.
This approach has been used in laboratory testbed to reach deep contrast levels, ten to the minus seven to ten to the minus ten, in support of future space-based missions. Deployment on ground-based systems remains challenging due to the dynamic nature of atmospheric turbulence, but has been successful in removing a fraction of static and slow speckles.
Sensing and compensation of non-common path aberrations can also be performed using a dedicated sensor, for example a Zernike phase-mask sensor.
2.3. Observing, Post-Processing, and Spectral Extraction Methods.
At sub-arcsecond separations, the raw, instrument delivered, planet-to-star contrasts obtained by the first generation of facility and now extreme AO systems, ten to the minus three to minus four point five, are still too shallow to yield decisive detections of many young Jovian exoplanets. Residual quasi-static speckle noise, due to nanometer-scale imperfections in telescope optics, thermal flexure, etc., limits instrumental contrasts and fails to simply average out over time like photon, white, noise.
Speckle noise statistics follow a modified Rician distribution whose long positive tail can lead to many false positives. Therefore, novel observing techniques coupled with advanced post-processing methods are critical to optimizing our ability to image exoplanets by further suppressing stellar halo light by orders of magnitude and making residual noise more Gaussian “whitening”. Various forms of differential imaging techniques are utilized to remove speckle noise for direct imaging. Commonly used techniques include:
Angular Differential Imaging, ADI.
ADI exploits the quasi-static nature of residual speckle noise in AO corrected high-contrast images from the ground and diffraction-limited imaging from space. For ground-based AO imaging, quasistatic speckles evolve on a characteristic timescale of 10 seconds to minutes to an hour or more. For space-based imaging, PSF breathing due to thermal variations and other small mechanical changes cause the quasi-static halo to evolve, but the halo for a given target can remain well correlated for months or years. By turning off the instrument rotator that keeps north fixed on the detector (on the ground) or obtaining observations at different roll angles (space), astrophysical objects off-axis from a star change position angle on the detector while the speckle halo remains fixed. Subtracting images obtained in an ADI sequence can then suppress speckle noise without fully suppressing planet signals.
ADI efficiently suppresses speckles on the ground and in space. It is the most widely used observing method in direct imaging.
ADI’s advantages are most limited at small angular separations where the displacement of the planet’s PSF is smallest for a given parallactic angle change or roll angle change. Typically, ADI induces signal loss, which must be corrected to achieve absolute spectrophotometric calibration for planets (see below).
Spectral Differential Imaging, SDI.
At raw contrasts shallower than the ten to the minus seven levels needed to image self-luminous Jovian exoplanets, achromatic phase errors dominate the wavefront errors responsible for the speckle halo. For data obtained simultaneously at multiple wavelengths for example dual-channel imaging or an integral field spectrograph (IFS), the speckle halo as a function of wavelength, scaled in radius by the central passband wavelength, is extremely well correlated. Since the images at different wavelengths are obtained simultaneously with the target, they do not suffer from are temporal decorrelation as with ADI. SDI then suppresses the speckle halo in a given passband by constructing a reference PSF drawn from (rescaled) images at other wavelengths.
Like ADI, SDI induces signal loss and is least effective at small angular separations, where bandpass rescaling yields small displacements of a (de-)magnified planet PSF. SDI’s efficacy is also limited by non-common path errors, differential sampling of the point-source PSFs across wavelength channels, and the spectrum of the planet to be detected. Extracting throughput-corrected spectra from data processed with SDI is also more challenging than with other differential imaging techniques.
Reference Star Differential Imaging, RDI.
RDI subtracts the PSF of a companion-less reference star from the target. It is widely used in the ultra-stable environment of space. While ADI and SDI may yield superior speckle suppression at moderate to wide separations, RDI may be advantageous at very small angular separations where other techniques suffer from self subtraction. RDI requires a very stable PSF to efficiently operate. Using it on ground-based telescopes may require fast switching between the target and reference star(s) for maximum contrast gain, in broad bandpasses, it also requires a reference star that is extremely well color matched.
These differential imaging techniques are used in combination with PSF subtraction algorithms. Many widely-used, advanced PSF subtraction methods are different forms of least-squares algorithms, which construct a reference PSF that minimizes the variance when subtracted from a given target image.
The locally-optimized combination of images (LOCI) algorithm constructs a reference PSF from a linear combination of reference images weighted by coefficients c determined from the solution to the matrix equation C equals A inverse times b, where A is the (square) covariance matrix for the reference library and b is the column matrix populated by elements multiplying each reference image by the target image. Another approach exploits principal component analysis (PCA), computing the Karhunen-Lo`eve transform of the reference image set and projects this set onto the target image to construct a combination of weighted eigen-images to subtract from the target (Karhunen-Loeve Image Plane algorithm, henceforth KLIP. Multiple successor algorithms such as TLOCI and A-LOCI draw from the lineage of LOCI and, or KLIP, employing advances such as correlation-based frame selection, pixel masking, various rank-truncations of the covariance matrix, free parameter optimization or exploiting high-performance computing to solve for the variance-minimizing coefficients directly.
Separate approaches involve maximum-likelihood methods to model and remove the stellar PSF or statistically modeling non-stationary covariances in small regions of images to improve PSF subtraction.
The relative performances of these algorithms vary in the literature and may depend on their exact implementation and suitability for a particular data set, although some work suggests that the newer algorithms descended from LOCI and KLIP can offer significant improvements. In general, all of these algorithms enable significant contrast gains over simple, classical methods, especially at small angular separations.
ADI, SDI, and RDI combined with PSF subtraction methods can attenuate and distort planet signals. The first methods to measure these biases and recover true planet flux measurements and astrometry injected synthetic planets into data at other locations or iteratively subtracted negative copies of planets at their apparent positions. Recently, several authors have developed efficient forward-modeling methods to estimate photometric and astrometric biases at the planet’s location. Planet forward models in turn can be used as matched filters to improve planet detection capabilities themselves.
Analyses of images whose speckle noise is partially suppressed by advanced PSF subtraction techniques have revised notions of how to quantify detection significances and spectroscopic uncertainties. For instance, finite sample sizes impact our definitions for contrast limits drawn from images with noise whitened by KLIP, LOCI, and other algorithms, especially at small, one to three lambda over D, separations. In IFS data, residual speckle noise may be spatially and spectrally correlated. Considering the full spectral covariance has a substantial impact on deriving planet atmospheric parameters from model comparisons.
Figure five shows the combined effect of high-contrast imaging hardware and software on imaging exoplanets, stepping through the successive improvements found from using extreme AO, coronagraphy, and PSF subtraction.
3. Direct Imaging Detections.
3.1. Taxonomy of Directly-Imaged Companions.
Figure one shows the demographics of planetary mass companions to stars detected via various methods. Community consensus on the planethood of many imaged objects, such as HR 8799 bcde, Beta Pictoris bc, 51 Eridani b, and so on, is clear. However, identifying the exact criteria needed to distinguish between planets and brown dwarfs is challenging.
Planets have often been identified as objects with masses below the deuterium-burning limit, nominally 13 times the mass of Jupiter, JUPITER MASS, the IAU Working Group Definition. However, this simple criterion is poorly motivated. In addition to being time and metallicity dependent, deuterium burning arguably does not identify a meaningful boundary for the evolution of low-mass objects, as some objects below the deuterium burning limit have been found in configurations which imply formation by cloud formation and other objects above the deuterium burning limit have been found in configurations which imply formation like a planet in a disk. For instance, recent imaging surveys have found objects that are members of quadruple systems, clearly formed by molecular cloud fragmentation, with inferred masses down to 5 Jupiter masses and free-floating objects with sub-deuterium burning masses as well.
In contrast, RV surveys have identified some systems, for example the 2.7 solar mass star nu Oph, with companions at around 150 Giga-meters, one astronomical unit, with masses of 22 and 24 Jupiter masses that are nevertheless locked in a mean-motion resonance indicating formation in a disk, meaning like a planet. The physics of planet formation does not require that gas accretion shuts off once 13 Jupiter masses of material is accreted.
An alternate definition leverages formation processes: a planet is an object formed in a circumstellar disk around a young star. Demographic analyses of substellar objects can provide empirically-motivated criteria for separating planets from brown dwarfs. Studies of the substellar mass function from previous RV surveys and recent ones, meaning the California Legacy Survey, show a local minimum at m sine (i) around 16 to 30 Jupiter masses.
The minimum in the companion mass function may be proportional to the primary mass, indicating that companion mass ratio (q) could be a key discriminator. Theory also suggests that the semi major axes (ap) and mass ratios (q) of companions also help distinguish between bona fide planets and brown dwarfs. Binary companions to more massive stars with q greater than about 0.025 are exceptionally rare.
Distributions of protoplanetary disk radii peak at around 200 au and fall to low frequencies by 30 Tera meters, 300 Astronomical Units.
Companions at wider separations are far less likely to have formed from a disk unless scattered to their current locations by unseen companions.
Thus, we set the following limits for a planet versus a brown dwarf:
Mass less than 25 Jupiter Masses, q less than 0.025, and ap less than about 45 Tera-meters (300 Astronomical Units.
Our linked spreadsheet lists the current inventory of directly imaged exoplanets, protoplanets, higher mass ratio, wider separation planet-mass companions, and controversial cases, hyperlink in text.
As of 19 October 2022, we identify 22 directly imaged exoplanets that fit our criteria, three of which are imaged protoplanets.
We also list another 34 dozen companions that may instead be better considered as brown dwarfs instead of planets, including companions orbiting brown dwarfs, and 6 “controversial” cases. Some of these individual classifications will undoubtedly change pending new analysis as may the exact values used to separate planets from brown dwarfs. Objects in each category will certainly be added over the next few years.
Below, we describe general properties of fully-formed directly imaged planets within 45 Terameters, 300 astronomical units and discuss three well-studied, emblematic cases, HR 8799, Beta Pictoris, and 51 Eridani. We also summarize our current knowledge of protoplanets and challenges with identifying imaged exoplanets.
3.2. Fully-formed Exoplanets.
3.2.1. General Properties.
Most directly imaged planets known thus far have near-IR contrasts ranging between ten to the minus four, kappa Andromedae b, and ten to the minus six, 51 Eridani b. All of them are self-luminous, made visible by thermal radiation released as the planets cool and contract.
On the sky, the planets generally lie at angular separations of point two to two seconds of arc, or about one to ten micro radians in grown up units, around 5 to 40 lambda over D for an 8m telescope.
Stars hosting imaged exoplanets typically have ages of 10 to 100 Mega years, many are found in young nearby stellar associations, often less than 100 parsec, 3,000 Peta-meters, with ages less than one hundred Mega years, that share similar kinematics (proper motions, space motions) and formed in the same star-forming region, such as Scorpius Centaurus or the beta Pictoris Moving group.
See Section 6.1 for more details on such associations as sites for exoplanet searches.
Most stars with imaged planets to date are B, A, or F stars, at least 50 percent more massive than the Sun. Most systems with imaged planets, including systems with the first detections, HR 8799, beta pictoris, also have Kuiper belt-like debris disks. Planets detected to date share similar spectral types and temperatures with more massive field brown dwarfs, but often show evidence of low surface gravity in their spectra. Brown dwarfs and exoplanets alike cool monotonically with age, beginning life as hot M type objects, cooling to the L spectral type (with very red near-IR colors and silicate condensate clouds, the cooler T spectral type (with blue near-IR colors and strong methane absorption at 1.6 micron and 2.2 micron, and eventually to the very cool Y spectral type.
Thus, there is an age, mass, temperature degeneracy for these objects, rendering mass estimates based on models very sensitive to the age assumed for the system. Most mass estimates for imaged planets depend on luminosity evolution models, challenges for which are described in Section 3.4. Some planets have dynamical mass measurements or limits. Masses inferred from luminosity evolution or derived from dynamics are typically around 5 to 10 Jupiter masses. Some planets have masses near the deuterium-burning limit, kappa Andromedae b, HD 206893 b. 51 Eridani b may have a far lower mass, as little as about 2 Jupiter Masses. Mass ratios for most imaged planets are q around 0.005 to 0.01.
51 Eridani b may have the lowest mass ratio, with q about 0.001. Even leading extreme AO systems are typically not sufficiently sensitive to detect young Jupiter-mass planets, let alone Saturn-mass planets. However, future capabilities will close these gaps (See Section 7).
3.2.2. Emblematic Systems.
While planetary mass companions to brown dwarfs had previously been detected as early as 2004 (most notably 2M1207b, the near simultaneous announcements of HR 8799bcd and Beta Pictrois b are widely regarded as the first bonafide directly-imaged exoplanet detections.
Planets in both systems were detected from ground-based facility AO systems in the near-to-mid IR in thermal emission, not scattered light. Just over 7 years later, Macintosh et al announced the first exoplanet discovered with extreme AO, 51 Eridani b.
HR 8799 is a nearby, around 40 parsec, 1200 Peta-meters, mid-A field star with an estimated age of about 40 Mega years.
The star hosts a massive, resolved Kuiper belt-like debris disk and a warm debris population interior to 1.5 Tera meters, ten Astronomical units consistent with an asteroid belt, possible signposts of massive, perturbing planets.
In 2008, Marois et al. announced the direct imaging discovery of HR 8799 bcd followed by a fourth planet discovery in 2010, HR 8799 e, located at projected separations of 2.25 to 10.5 Tera meters, 15 to 70 astronomical Units, Figure 6, left panel.
Soon after HR 8799 bcde’s announcements, other studies identified one or more planets in archival or separately obtained data.
A decade of Keck Observatory monitoring showed that HR 8799 bcde orbit close to the 1 to 2 to 4 to 8 resonance.
Current analyses suggest that the planet orbits are nearly coplanar with the disk, with a small inclination of about 27 degrees. However, HR 8799 e may not orbit on a plane strictly coplanar with HR 8799 bcd
Originally, masses inferred from the planets’ luminosities spanned a wide range of values, 5 to 13 Jupiter masses. However, dynamical stability modeling strongly favors masses below 10 Jupiter Masses, 7 Jupiter Masses for HR 8799 cde, HR 8799 b.
Using Hipparcos and Gaia, measured a dynamical mass for HR 8799 e of about ten Jupiter masses, consistent with these limits, see Section 3.5. Additional planets may explain the shape of the inner edge of HR 8799’s cold belt, especially if they are near or below Jupiter’s mass.
Any planets interior to HR 8799 e must be below three or four Jupiter masses at one to one point five Tera meters, 7 to 10 Astronomical Units, and five to six Jupiter Masses at six hundred to a thousand Giga-meters, or 4 to 7 Astronomical Units.
The HR 8799 planetary system resembles a scaled-up version of our own outer solar system.
HR 8799 bcde have been benchmark objects for understanding the atmospheres of young Jovian planets. Their photometry and low-resolution spectra differ from those of older, field substellar objects thought to have similar temperatures, identifying features diagnostic of clouds, chemistry, and gravity. Higher resolution spectra probe molecular abundances connected to formation mechanisms.
Section 4 discusses HR 8799 bcde’s spectra and atmospheres in more detail.
Beta Pictoris.
The A6V star Beta Pictoris is the eponymous member of a collection of kinematically-associated stars known as the Beta Pictoris Moving Group, with an age of around 20 million years. The star hosts an edge-on debris disk, imaged since the mid-eighties from the ground and space.
The disk consists of a planetesimal ring at ten to 15 Tera-meters responsible for the bulk of the dust through collisions and an outer extension comprised of small dust grains blown out by radiation pressure.
Lagrange et al. first identified Beta Pictoris b from data obtained in 2003 with VLT, NaCo; follow-up data obtained one year later confirmed the planet.
Thanks to Beta Pictoris’ b’s small semi-major axis 1 point 35 Tera-meters, or around 9 Astronomical Units, over 75 percent of its orbit has been monitored.
More recently, a second planet Beta Pictoris c was identified from RV data and then recovered with high contrast inteferometric imaging with VLTI, GRAVITY.
Beta Pictoris b may partially explain the observed warp in the edge-on debris disk and the many evaporating exocomets identified over the past 30 years.
Planets besides Beta Pictoris b c could explain why the inner 70 Astronomical Units, 10 Tera-meters is relatively devoid of debris dust; localized features in the disk also suggest additional planets could be present. From RV and astrometric data estimate a mass of 9.3 and 8.3 Jupiter Masses for Beta Pictoris b and c respectively.
Although these values heavily depend on the assumed uncertainties on the RV data. RV and direct imaging data combined exclude additional planets more massive than 2.5 JUPITER MASS from 15 Giga meters to Tera meters, 0.1 to hundreds of Astronomical Units.
Beta Pictois b is more luminous and hotter than the HR 8799 planets, effective temperature 1700 to 1800 Kelvin, just slightly redder than field early L-type dwarfs, and is likely cloudy, dusty with a low gravity.
Beta Pictois c probably has a temperature intermediate between its sibling and the HR 8799 planets.
51 Eridani.
51 Eridani is a 29 parsec,900 Peta meter, distant early F star, a member of the about 20 million years old beta pictoris Moving Group, and a member of a wide hierarchical triple system that includes an M dwarf binary GJ 3305. 51 Eridani has a detected infrared excess, modeled with a cold dust belt located approximately between 750 to twelve thousand Giga-meters, 5 to 80 Astronomical Units.
The GPI campaign (GPIES) team discovered 51 Eridani b, a faint planet at projected around 2 Tera-meters, 13 Astronomical Unit, Figure 6, right panel.
The planet is the first discovered using extreme AO and the first incontrovertible T dwarf planet, showing strong methane absorption in H band. The planet is likely more eccentric than either beta pictoris b or HR 8799 bcde.
If confirmed, an eccentric orbit could indicate the presence of an additional massive body or could be due to gravitational perturbations from GJ 3305AB. Assuming a hot-start luminosity evolution, current data rule out other planets more massive than 4 JUPITER MASS beyond 5 Astronomical Units and more massive than 2 JUPITER MASS beyond 9 Astronomical Units.
The mass of 51 Eridani b is not well constrained: values derived from comparing 51 Eridani b’s luminosity and age to evolutionary models favor about 2 JUPITER MASS, while atmospheric modeling may favor larger values, up to about 9 JUPITER MASS .
Different characterization studies also find slightly diverging atmosphere properties, illustrating the challenge associated with characterizing very faint exoplanets with direct imaging
Analysis of Gaia and Hipparcos astrometry set an upper limit of 11 JUPITER MASS for 51 Eridani b
The planet likely has a temperature of around 700 Kelvin and either lacks clouds or is only partially covered by clouds.
3.3. Protoplanets.
Direct images of planets in active assembly, protoplanets, around stars that still retain gas and dust-rich protoplanetary disks clarify how and where planets form. The large distances to the nearest star-forming regions, around 150 parsecc, mean that protoplanets orbiting their host stars at solar system scales are located at very small angular separations.
However, protoplanets can be bright, Luminosities around a hundredth to a thousandth of the solar luminosity, especially if they are surrounded by their own circumplanetary disks.
The 5 megayear old 0.87 solar mass star PDS 70 hosts the first incontrovertible detections of Jovian protoplanets: PDS 70 b and PDS 70 c.
Both protoplanets are located within the PDS 70 disk cavity, at angular separations
Point eighteen and point twenty four arc seconds and estimated semimajor axes 20 and 34 Astronomical Units, 3 and 5.1 Tera-meters.
Dynamical arguments strongly favor masses less than 10 JUPITER MASS for PDS 70 b, while PDS 70 c’s mass is more poorly constrained. Masses inferred from SED modeling range between 1 and a few Jovian masses.
PDS 70 b is slightly eccentric, eccentricity 0.17, while PDS 70 c’s orbit is consistent with being circular. The protoplanets’ IR data are best fit by model atmospheres with substantial dust, extinction.
PDS 70 bc show H alpha emission consistent with accretion at rates of one to two solar masses per hundred million years, slightly less than the stellar accretion rate, but lack evidence for Br gamma accretion.
PDS 70 c shows direct evidence for a circumplanetary disk with an estimated mass of 0.007 to 0.03 Earth Mass. Thermal IR data may suggest that PDS 70 b is surrounded by a circumplanetary disk.
Recently, data from Subaru, SCExAO and the Hubble Space Telescope over 13 years reveal evidence for a wide separation, 93 astronomical unit embedded protoplanet around the one to three million year old, 2.4 solar mass star AB Aurigae.
AB Aurigae b is consistent with a protoplanet responsible for the millimeter dust cavity and CO gas spirals both seen by ALMA. It appears spatially extended, plausibly due to light from the central source reprocessed by the star’s protoplanetary disk. The best-fit composite model explaining AB Aurigae b’s optical to near-IR emission includes a 2.75 Jupiter Mass, 9 Jupiter mass source with a surface gravity of log(g) equals to 3.5, emitting at a much hotter temperature than PDS 70 bc, around 2000 to 2500K, and accreting at a rate of one point one Jupiter masses per million years. The source is detected in H alpha although it is unclear whether this emission is due to accretion. Embedded in a massive disk with numerous spiral arms at over three times Neptune’s disk instead of in a fully cleared cavity like PDS 70 bc, the properties of AB Aurigae b may point to a planet formation mechanism by disk instability (see Section 6).
Prior to the discovery of PDS 70 bc, other studies claimed detections of protoplanets located within the gaps of or embedded in disks around young stars. The 2-solar mass, protoplanetary disk-hosting star HD 100546 has a protoplanet candidate at around 7.5 Tera meters, 50 astronomical units and another at around 2 tera meters, 13 astronomical uunits, just interior to the gap in the protoplanetary disk (HD 100546 bc.
HD 100546 b has been detected in multiple data sets but evidence for orbital motion is not yet clear.
HD 100546 c has been imaged in a single data set and inferred through spectroastrometry but not yet imaged in subsequent data.
Interpreting both candidates, whether a planet or disk feature, is challenging.
Both candidates require further study and confirmation.
Two studies presented detections of protoplanets around the young, Sun-like star LkCa 15 through a combination ofsparse aperture masking interferometry (SAM) and H alpha differential imaging, LkCa 15 bcd.
However, later direct imaging observationsshowed that the SAM detections correspond to disk features LkCa 15 b technically remains a candidate for now due to its single epoch H alpha. Other claimed protoplanet detections have been revealed to likely be misidentified disk signals instead of planets.
3.4. Challenges with Interpreting Detections.
3.4.1. Confirming Companionship.
Direct imaging observations reveal many point sources that are unrelated background stars instead of bound companions, especially for systems in the Galactic plane. Confirming candidate planets as bound, meaning sharing common proper motion with and orbiting their stars, often requires multi-year observations, depending on the star’s proper motion. In some pathologicalcases, background stars can have a non-zero proper motion and thus are more easily confused with bona fide planets.
In the absence of full confirmation, an object’s near-IR spectrum can provide strong evidence that it is a directly-imaged exoplanet, for example for 51 Eridani b.
3.4.2. Estimating Accurate Masses.
Masses for imaged planets and planet candidates are typically not directly measured but are instead estimated using luminosity evolution models that map between an object’s brightness and mass as a function of age. Accurate mass estimates therefore are affected by uncertainties in these models and require precise system ages. Hot start models for planet luminosity evolution assume a high initial entropy, resulting in bright planets for the first one to a hundred Mega years.
Cold start models assume a low initial entropy, resulting in planets that are substantially fainter for the first 100 Mega years.
Originally, hot start models were used to describe planets formed by disk instability, while cold start models described planets formed by core accretion.
However, recent models show that core accretion formed planets can be compatible with hot start-like luminosity evolutions, and Jovian planets may form with a range of initial entropies.
Aside from 51 Eridani b, all planets imaged thus far are only consistent with a hot-start luminosity evolution.
Precise ages can be exceptionally challenging to derive for isolated stars.
Members of young moving groups or other nearby associations can be age-dated using a variety of methods: for example fitting group Hertzsprung-Russell (HR) diagram positions with stellar evolutionary models, Lithium abundances. Thus, moving group or association membership can yield a star’s age.
However, even stars with a motion similar to bona fide moving group members could instead be interlopers, especially if they have dissimilar space positions. For isolated Sunlike stars, stellar rotation and activity can give age estimates, albeit with significant scatter.
For early-type stars, HR diagram positions have provided approximate ages: optical interferometry can now provide more precise estimates by resolving the stars themselves.
Revisions in the stellar age often affects the interpretation of an imaged companion. For example, based on the primary’s claimed membership in the Columba association, Kappa Andromedae b was originally thought to be 12 to 13 JUPITER MASS.
In contrast, the primary’s HR diagram position resembles an older system, 220 Mega-years, which would imply the companion is around 50 JUPITER MASS.
However, through optical interferometry, it has been shown that the star is likely (nearly) coeval with Columba even if it is not a member around47 Mega years, implying the object is a planet mass companion. Later, near-IR spectra of Kappa Andromedae b were found to be consistent with a planet interpretation.
In contrast, the imaged companion to GJ 504 was announced as a 3 to 8.5 JUPITER MASS planet with an age of 100 to 510 Mega years.
Based on interferometric, RV, and high contrast imaging data, derived a mass range of 1 to 23 JUPITER MASS, while other analysis of the star suggested an age of about 2.5 Gyr and a mass well into the brown dwarf regime.
3.4.3. Planet or disk feature?
Distinguishing between highly-structured disk signals and bona fide protoplanets is a steep, chronic challenge.
Advanced algorithms needed to detect protoplanets can attenuate both disk and planet signals: forward-modeling is required to ensure that a claimed planet detection is not a partially subtracted piece of the disk.
For systems with hot dust, T around 1000 to 2000 K, near the star that intercepts and reprocesses emission, the disk’s scattered light spectrum may strongly resemble spectra of bona fide protoplanets. Orbital motion over multi-year timescales can better establish that a signal is an orbiting planet and not a static disk feature H alphaission can also pinpoint actively accreting protoplanets however, accretion onto protoplanets embedded in disks may be unidentifiable, since optical extinction likely renders Hα undetectable.
Fomalhaut b may represent the first of yet another class of objects with a challenging interpretation
The object was initially identified as a directly imaged planet, made visible by both reflected light from a circumplanetary disk and thermal emission, and responsible for sculpting the star’s Kuiper belt-like debris disk. However, later analysis showed that Fomalhaut b’s spectrum is completely explained by scattered starlight and its orbit likely crosses the ring the object is lower in mass and likely made visible purely by circumplanetary dust. Recently, from analyzing archival and unpublished data, Gaspar and Rieke (2020) proposed that Fomalhaut b may be fading and dispersing, consistent with a massive planetesimal collision. Other analyses find no clear evidence for these two trends, although they were conducted only over a subset of available data. Future observations of Fomalhaut b and, or reanalyses of recent data may clarify its true nature.
3.5. Synergies with Other Techniques.
The limitations of direct imaging affect our ability to interpretdata for individual objects and to draw conclusions from large-scale population studies. For individual objects, mass estimates depend on both the age of the planet and the chosen luminosity evolutionary model (see Section 3.4). At a population level, the angular resolution of the telescope and the achievable contrast of the instrument limit the range of detectable planetary masses and semi-major axes with direct imaging. Combining direct imaging with other planet detection techniques partially mitigates these limits.
Optical interferometry provides a means to directly detect bright, modest planets at small angular separations.
As a prime example, GRAVITY interferometer coherently combines the light of all four VLT telescopes, yielding the equivalent angular resolution of a 130-m telescope, GRAVITY. While the GRAVITY field-of-view is small (around 50 mas), indirect detection techniques, such as RV, cna help predict the position of an exoplanet candidate. GRAVITY has yielded exceptionally high-SNR spectra and ultra-precise astrometry of known planets, the combination of GRAVITY and indirect techniques have now resulted in new planet discoveries
Relative astrometric measurements of a planet around mass of the orbiting planet; the semi-major axis of the relative orbit encodes the system’s total mass, not the individual components’ masses. The system’s mass ratio, and thus the mass of the planet, can be measured if the semi-major axis of the orbit of the star around the system barycenter is known. This orbit can be measured either using absolute astrometric measurements from catalogues such as Hipparcos and Gaia, or spectroscopic observations to measure the Doppler shift of the star’s spectral lines over the course of the orbit. In some cases, high-resolution spectroscopy can measure the Doppler shift of the planet’s spectral lines, also yielding the mass ratio. Combining the star’s orbit around the barycenter with the relative astrometry between star and planet yields the mass ratio, and a dynamical mass for the planet. In multi-planet systems with high-precision astrometry, the planets’ mutual gravitation can be detected by fitting for deviations from Keplerian motion, yielding their mass estimates.
The Beta Pic system is the current benchmark example of combining planet detection techniques to measure model independent masses. Relative astrometry of Beta Pic b and c combined with the star’s astrometric and RV measurements provides dynamical masses for the planets
Measurements of non-Keplerian motion due to the mutual gravity of Beta Pic b and c are also now possible given the precision of recent interferometric monitoring campaigns.
Combining astrometry and, or RV and direct imaging data can yield dynamical masses for other directly-imaged planets, as well as for numerous brown dwarf companions.
The upcoming Gaia data releases for the full and extended mission will be used extensively in future detection and characterization studies of directly-imaged planets, yielding further dynamical masses for directly imaged companions. The extended mission will provide precision astrometric measurements of the host star over around 10 years.
Combining direct imaging with other detection techniques can improve planet occurrence rate measurements and can better determine the planet frequency distribution over a range of masses, orbital periods, and host star properties. Long-term Doppler surveys are largely complete to giant planets within about 750 Giga-meters, 5 astronomical units, and partially complete to giant planets out to around10 astronomical units, the current inner limit of sensitivity of direct imaging surveys. Thus, combining results from both techniques reveals giant planet demographics out to around100 astronomical units (Section 6.3). RV studies of young stars allow occurrence rates to be compared for stars of similar ages, tracing the extent of giant planet migration over system lifetimes and its effect on planet frequency. The final Gaia planet catalog will also help place demographic measurements of young, wide-separation giant planets into context, as astrometry will be better able to probe intermediate separation giant planets.
150 to 1,500 Giga meters, around one to ten Astronomical units, Masses greater than around one Jupiter masses, around younger, higher-mass stars compared to RV. These target stars more closely match the hosts of imaged planets. A Gaia-selected survey of accelerating stars has now led to the first joint direct imaging and astrometry discovery of an exoplanet.
Combining Gaia observations of younger, higher-mass stars with direct imaging will illuminate trends in occurrence rate as a function of stellar mass and age.
Direct imaging also shares an important overlap with planet detection by microlensing. Both techniques are sensitive to planets beyond the snow line, with microlensing probing lower planet-star mass ratios, while the host mass of imaged planets can be directly determined, and the planet mass inferred from models.
In addition, singly lensed short-period microlensing events can represent either a free-floating planet or a wide separation bound planet. Constraints on the wide-separation giant planet population can be determined from imaging, thus more definitively constraining the free-floating planet occurrence rate.
4. Atmospheric Characterization of Directly Imaged Exoplanets.
Direct imaging enables characterization of young, age less than 200 Mega years Jovian exoplanets at wider separations with negligible irradiation compared to most transiting planets. In this section, we first summarize from an empirical standpoint what we have learned about the atmospheres of the current cohort of young directly imaged giant planets from their photometry and spectroscopy. We then consider the theoretical side of the picture, in particular, the state-of-the art in how we model these complex, cool atmospheres.
4.1. Empirical Constraints from Time-Averaged Observations.
4.1.1. Photometry.
Photometry in the major near-to-mid IR passbands, J, H, K, Lp, Ms, covers the bulk of emission for young super Jovian planets and provided the first empirical diagnostic of young, directly-imaged planet atmospheric properties. Figure 8 displays a typical near-IR color magnitude diagram for selected directly-imaged planets and other planet mass companions compared to older, more massive field brown dwarfs and younger brown dwarfs whose ages and masses partially overlap with those of most imaged planets.
Imaged planets and planet-mass companions span the full luminosity range characteristic of mature late-M, L, and T field brown dwarfs (vertical axis). However, young brown dwarfs, planets, and planet-mass companions typically have redder colors than field objects.
The most pronounced differences between planet and methane-poor L-type dwarfs to methane-absorbing T-type dwarfs. The first imaged planet-mass companion, 2M 1207 B, and some of the first imaged exoplanets, for example, HR 8799 b) are particularly discrepant, appearing to populate a previously empty part of these diagrams consistent with a reddened extension of the L dwarf sequence to lower luminosities.
Over the full 1 to 5 micron spectral range, photometry for these objects appears redder and more blackbody-like, Figure 10, top-left. More recent studies show that a number of other young imaged planets, planet-mass companions, for example HD 95086 b, TYC 8998-760-1 c, HD 206893 b, 2M 2236 plus 4751 B, also populate this region.
Differences between field and young objects with T spectral types are less clear. Few young, T-type planet-mass companions have been identified in the last decade, for example 51 Eridani b.
While GU Piscium B follows the sequence of field brown dwarfs in near-IR color-magnitude diagrams, the exoplanet 51 Eridani b is redder than field brown dwarfs. Four additional companions orbiting primary stars with intermediate ages, less than around one Giga-years.
HN Pegasi B, ROSS458C, BD204-39B, and others, show similar but less pronounced deviations with respect to field dwarfs in the same luminosity range. Some directly imaged L, T transition planets and planet-mass companions have color-magnitude diagram positions discrepant from field objects in thermal IR passbands probing methane absorption (3.3 microns, Ms) but have positions similar to field objects in other passbands.
4.1.2. Spectroscopy.
Over the past decade, IFS instruments, especially those used in combination with extreme AO systems (P1640, SPHERE, GPI, SCExAO-CHARIS) have provided critical low-resolution (R around 20 to 80) near-IR, 1 to 2.5 micron spectra of most directly-imaged exoplanets, a representative sample of which is displayed in Figure 9. Near-IR spectra provide key diagnostics of brown dwarf and planet atmospheres. Spectral indices derived from low-resolution data like H20 yield coarse estimates of spectral types.
The H-band continuum index and (possibly) the H2-K index is a diagnostic of surface gravity.
Following trends from photometry, the spectra of young directly-imaged planets and other planet-mass companions show significant differences with the spectra of field brown dwarfs. Among the most notable are the following:
Chemistry. The L, T transition traces the onset of methane absorption. However, spectra of some L, T transition exoplanets and planet-mass companions, for example the HR 8799 planets, 2M 1207 B, show a lack of methane absorption compared to field dwarfs with similar temperatures and CMD positions.
Thermal IR spectra confirm that the HR 8799 planets also have weaker absorption in the 3.3 microns methane filter.
Gravity. The H-band spectra for L dwarf and L, T transition directly imaged exoplanets, planetarymass objects such as HR 8799 b, Kappa Andromedae b, ROXs 42Bb show highly peaked H-band spectra and, or red K-band spectra compared to field dwarfs. The H and K-band shapes probe collisionally-induced absorption (CIA) of hydrogen; lower gravities result in weaker CIA and thus peaked H-band peaks and redder K-band slopes.
Dust. At least some of the HR 8799 planets have spectral properties and molecular absorptions that are well matched by those of young free-floating objects at the L, T transition (see also Section 4.3). However, no object yet reproduces the available 1 to 2.5 micron spectra of HR8799b, which may be due to the lack of identified young free-floating T-type objects.
A handful of early-T-type objects such as the AB Doradus member 2MASS, number in text, can reproduce the spectral bands of HR 8799 b provided that an extra layer of extinction by sub-micron dust particles is applied to these empirical template spectra to match the spectral slope of the planet.
HD 206893 b presents an even more extreme example, with an even flatter, more blackbody-like spectrum yielding an extremely red spectrum from 1 to 2.5 micron, likely due to substantial atmospheric dust.
Spectra of the coolest and lowest mass imaged exoplanet known to date, 51 Eridani b, display a clear and so-far unique detection of a methane absorption at 1.6 micron in the spectrum of an imaged exoplanet, coincident with an enhancement in the K-band flux.
Similar K-band flux enhancements have already been noted in young mid to late-T dwarfs but the enhancement is particularly extreme in the case of 51 Eridani b and is due to the reduced collision induced absorption of H2 in 51 Eridani b’s lower-pressure atmosphere.
Protoplanets display far more featureless, black bodylike spectra. PDS 70 bc’s spectra reveal an extremely red spectral continuum devoid of the strong water-band feature, 1.3 to 1.4 micron expected given the observed luminosity of the planets.
Higher resolution K-band spectra show a lack of molecular absorption in PDS 70 b’s spectrum.
The circumplanetary disks and, or cocoon surrounding each planets, see Fig 7, produce significant foreground extinction and may produce spectroscopic properties similar to what is seen in the near-infrared spectra of enshrouded class one protostars. The near-IR spectrum of AB Aurigae b is reproduced by a 2000 to 2500 Kelvin blackbody but likewise lacks clear evidence for molecular absorption common in fully-formed substellar objects with similar temperatures.
Aside from a few isolated cases, for example HR 8799 bcde, the spectroscopic properties of imaged exoplanets are more poorly constrained at wavelengths longward of 2.5 micron. The 3-5 micron range is particularly interesting since it contains both methane and 12 Carbon Monoxide absorption features diagnostic of carbon chemistry, Carbon Monoxide, Methane and cloud structures.
The Lp-band spectra of hotter young M7 to L3 companions, show no significant difference with those of field dwarf counterparts. The Lp spectrum of kappa Andromedae b is well matched with that of yo
-
1:29:26
Glenn Greenwald
13 hours agoICE Detains Permanent Resident for Protesting Israel; European Leaders Make Maniacal Rearmament Vows They Cannot Keep | SYSTEM UPDATE #421
146K280 -
1:02:56
Donald Trump Jr.
15 hours agoUSAID Slush Fund Slashed, X Cyberattack, Plus Interview with Nate Morris | Triggered Ep.223
140K165 -
5:48:40
Dr Disrespect
19 hours ago🔴LIVE - DR DISRESPECT - THE SHOTTY BOYS - WARZONE, PUBG, FORTNITE
247K43 -
2:12:50
Adam Carolla
20 hours agoDouble Murder Convict to be executed by Firing Squad + Comedian Elon Gold + Comedian Carol Leifer
98.6K21 -
46:08
Kimberly Guilfoyle
16 hours agoBad Day to be a Bad Guy: FBI Taking Down World’s Worst Criminals, Live with John Nantz | Ep.203
204K85 -
DVR
Redacted News
14 hours agoWhat's REALLY going on in Syria? | Redacted with Natali Morris
203K135 -
54:18
Candace Show Podcast
15 hours agoHarvey Speaks: Jessica Mann & The Five Year Affair | Ep 3
221K120 -
56:53
Grant Stinchfield
14 hours ago $10.19 earnedFreeze Spending & Kick the Can Down the Road... Why Republicans Should do Just That!
120K25 -
56:48
VSiNLive
14 hours agoFollow the Money with Mitch Moss & Pauly Howard | Hour 1
88.6K1 -
3:28:27
Barry Cunningham
15 hours agoTRUMP DAILY BRIEFING: INTERNET UNDER ATTACK! X & RUMBLE DOWN! EXECUTIVE ORDER SIGNING!
107K62